Loss of heterogeneity, quiescence, and differentiation in muscle stem cells
Satellite cell heterogeneity
Muscle growth during embryogenesis, and muscle homeostasis and replacement upon injury in the adult rely on muscle stem cells, referred to as satellite cells. Satellite cells are quiescent and become activated following injury. Activation results in extensive cell proliferation (proliferating satellite cells are called myoblasts), followed by myogenic differentiation and fusion of differentiated cells into myotubes and then myofibers that repair the muscle. During myotube formation, a small fraction of satellite cells self-renews and returns to the quiescent state, thereby providing a reservoir for future muscle repair. A single muscle myofiber contains multiple nuclei (up to several hundred in mice), which result from fusion of differentiated myogenic cells and they are post-mitotic. Adult myogenesis involves all phases of the cell cycle, and also a reversible (satellite cells) and irreversible (post-mitotic myogenic cells) cell cycle exit (Figure 1). In the embryo, where stem cells continually yield differentiating cells to ensure tissue genesis, muscle stem cells proliferate and they enter quiescence during the perinatal period in the mouse (1), therefore, these cells essentially explore cell cycle progression.
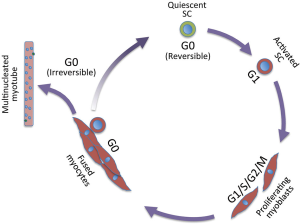
Muscle stem cells are defined by transcriptional programs that rely on both Pax3 and Pax7 transcription factors, the former with a prevalent role in the embryo, and the latter from mid-embryogenesis onwards [for a review, see (1)]. Myogenic regulatory factors (MRFs) Myod, Myf5, Mrf4, and later Myogenin act sequentially to promote satellite cells commitment and differentiation. Although muscle stem cells are well characterized by the abovementioned transcription factors, heterogeneity of both embryonic and adult stem cells has been reported extensively [for reviews, see (2,3)]. In the embryo, the ancestors of satellite cells display heterogeneity in behavior including different proliferative rates (4), requirement for upstream specific transcription factors [e.g., Pax3 is not required for head muscle progenitors (5); extraocular and pharyngeal arch muscle progenitors rely on distinct regulatory factors (6)], commitment transcription factors [Myf5 and Myod (7); see also (1)]. Muscle stem cell heterogeneity persists in the adult where satellite cell heterogeneity has been reported for transcription factors and surface markers [for a review, see (2)], type of muscle (8), transplantation efficiency (9) metabolism (10), and age-dependent loss of quiescence and self-renewing capacity (11).
Moreover, subpopulations of satellite cells in adult mice (all express Pax7) have been identified. For example, during homeostasis and regeneration Pax7Hi cells display more “stemness” properties, with higher capacity for asymmetric division and self-renewal during the regeneration process (10). A subpopulation of “dormant” Pax7+ cells has been also reported with capacity to survive and maintain full regenerative capacity in necrotic tissues after death in mice and humans (12). It remains unclear to what extent the expression levels of Pax7 correlate with post-mortem “dormant” satellite cell populations. Pax7Hi cells from homeostatic muscle and those isolated from post-mortem tissue share several properties including reduced mitochondrial and metabolic activity. Heterogeneity is also evidenced in the decline of regenerative capacity as most satellite cells in old mice (20–24 months of age) undergo permanent cell cycle withdrawal via senescence that blocks their proliferation capacity (13). This capacity is however restored in a subset of cells in the presence of rejuvenation factors (14), whereas this is not the case in geriatric mice (28–32 months of age). Nevertheless, in geriatric mice a subpopulation of cells re-enter the cell cycle via induction of autophagy (15). Thus, in adult mice, satellite cells are heterogeneous also in the context of quiescence and cell-cycle exit [see also (16)].
The origins and the function of this heterogeneity are not clear, but it is believed that it endows satellite cells with a level of plasticity that is necessary to adapt to different needs of skeletal muscle growth, homeostasis, regeneration (3), and resistance to unfavorable conditions. More generally, stem cell heterogeneity is expected to maintain a range of functional capacities to face changing conditions and be operational under a variety of types of stress. It is thus counterintuitive that, at least under some conditions, muscle growth and regeneration would rely on the loss of satellite cell diversity and accentuate the proliferation of single satellite cells. Since heterogeneity as well as its loss are linked to the switch between quiescence/re-entry in the cell cycle and proliferation/differentiation, this condition is essential for understanding stem cell properties as well as their regenerative potential.
The clonal drift
The “clonal drift” model implies expansion of clonally related stem cells and has been originally reported for tissue replacement by intestinal stem cells in the adult and later in other tissues and organisms. Unlike other mammalian tissues, the stem cells of the intestine are strictly compartmentalized in crypts, and the cellular progeny remain associated with the stem cell compartment of origin. Crypts then drift toward clonality. According to the prevalent model, adult intestinal stem cells (Lgr5hi) divide symmetrically, and stochastically one of them adopts a stem cell fate (the other proliferates/differentiates following transit amplification) (17). This process results in the stochastic loss of stem cell properties in one case, and expansion of the progeny in the other, eventually leading to a pool of clonally related stem cells that replenish the entire stem cell population. At the population level, clones expand and contract at random until they either take over the crypt or are lost, and finally stem cell loss is compensated by proliferation of the neighbor, with a concomitant increase of clonality (18). This mechanism is retained also for homeostasis of the fish intestine (but not in other tissues), suggesting that the mode of stem cell division is not species-specific but is instead characteristic for the stem cell type (19). In an experimental paradigm in the mouse, it has been shown that when competition involves wild type versus mutant cells with an accelerated division rate (dependent on the oncogene K-ras), this process results in clonal expansion of crypts carrying the oncogenic mutation, with potential implications for cancer development (20).
Stem cell loss and replacement, apparently at random, has been also shown as a mechanism for niche occupancy of epithelial follicle stem cells (FSCs) in the Drosophila ovary during normal homeostasis (21). In this case, establishment of cell polarity is an important early differentiation event and FSCs that maintain immature polarity have an advantage in occupying the niche. Reduced clonal complexity leading to clonal drift in muscle stem cells has been recently reported only following successive injuries and tissue repair events in adult mice, but not during ageing (22). This impoverishment of stem cell diversity, however, did not lead to an apparent reduced regeneration capacity, at least in young mice. In other contexts, reduction of the clonal diversity has been associated with non-beneficial outcomes. For instance, somatic mutations drive clonal expansion of cells in tumors. However, the size of clonal expansion does not necessarily correlate with the capacity of inducing malignant transformation in skin (23), and somatic mutations leading to clonal outgrowth in hematopoietic cells are frequent in the general population, the majority of which does not develop blood cancers (24).
Clonal drift has been poorly investigated during embryogenesis. This condition is of particular interest because during organ growth there is a continuous need for cellular differentiation and repopulation of active stem cells. Some studies suggest that clonal dominance is a recurring mechanistic strategy to help shape vertebrate organs. In zebrafish, it was reported that about eight dominant cardiomyocytes in the embryonic ventricle contribute to building the adult cortical myocardium (25), and more generally a few lineages contribute to the formation of most adult organs (26). A recent study by Nguyen et al. (27) directly addresses this topic, and also provides a mechanism that drives clonal drift in the developing muscle in zebrafish. Using a muscle-specific fluorophore maturation assay that relies on two fluorophores with different maturation rates, they identify fiber ages during growth, and combine this analysis with morphometric measurements. They show that muscle stem cells undergo clonal drift, and the majority of muscle fibers in the adult are generated from a limited number of stem cells. Thus, initially multiple, independent stem cells contribute to fiber generation, whereas after successive self-renewal events, a single stem cell clone dominates growth in individual myotomes. As in the cases of homeostasis in the adult described above, also homeostasis during muscle growth appears to occur randomly. Importantly, Nguyen et al. showed that the clonal drift is controlled by the homeobox protein Meox1 that inhibits the cell cycle checkpoint gene ccnb1 halting stem cells in G2 phase, whereas the other cells undergo proliferation and differentiation (Figure 2).
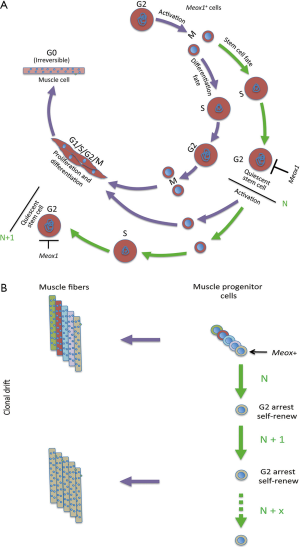
Meox1 is a homeobox transcription factor that together with Meox2 regulates the development of somites that give rise to the sclerotome, dermomyotome and myotome (28). Meox1 impairment alone affects the sclerotome. Meox1 also plays a role in the commitment of the skeletal muscle lineage (29). Interestingly, in endothelial cells Meox1 and Meox 2 arrest the cell cycle at the G1/S transition through activation of the cycline-dependent kinase inhibitors p21CIP/WAF1 and p16INK4 (30), where overexpression of these proteins is a known factor leading to cellular senescence (31).
Two notable points emerge from the Meox1-dependent clonal drift study in the zebrafish myotome. First, the determination of cell fate by direct cell cycle arrest rather than induction of cell cycle exit by exogenous factors. As examples of exogenous factors: non-acquisition of polarity in one of the daughter cells determines the stem cell fate in FSCs; or positional or niche-dependent factors are assumed to be determinant in much of the random events described above. Direct regulation by a single transcription factor has the advantage of effecting a direct response by regulation of a specific target gene. However, one cannot exclude that exogenous factors contribute to the cell fate decision in the zebrafish myotome, since loss of segmentation and abnormal skeletal organization may contribute to the loss of the clonal drift in meox1 mutants. Second, the transient cell cycle arrest in the G2 phase, which contrasts with a transient arrest in G0 that is frequently observed during quiescence (32). Quiescence is a non-proliferative, metabolically resting phase displayed by many adult stem cells and by some organisms in response to nutrient limitations or adverse survival conditions. Quiescence in G0 occurs in cells whose genome has not yet replicated, whereas muscle stem cell quiescence in the zebrafish embryo, which is correlated with the maintenance of stemness, occurs in G2 and therefore in 4n cells.
G2 pausing has been associated with efficient adult stem cell regeneration in a variety of organisms. For instance, adult stem cells of Hydra, a freshwater invertebrate with high regenerative potential, display a robust pause in G2, which can be maintained over weeks of starvation, and this is correlated with vigorous regeneration (33). Similarly, regeneration of amputated limbs in the Mexican salamander (Axolotl) depends on upregulation of Evi5, a centrosomal protein that regulates the G2 arrest by preventing mitosis. This last mechanism is shared with skin regeneration (ear hole punches) in super-healing MRL mice (34), which also display downregulation of p21 (a key trigger of senescence).
In differentiated cells, G2 arrest has been generally associated with the response to DNA damage to allow time for DNA repair. Since the DNA is fully duplicated in this phase, repair of DNA double strand breaks (DSBs) that might result from the replication process itself can rely on the presence of the sister chromatid and therefore on homologous recombination. In these cases, the cell cycle resumes upon repair of the DNA damage. However, if DNA damage is too extensive, or repair is inefficient, DNA damage accumulates and prolonged G2 arrest may become incompatible with the proliferation requirements of the cell, leading to cell death. Alternatively, the cell cycle arrest becomes irreversible leading to cell senescence. Overcoming the G2 blockage in the presence of DNA damage may drive the cell to apoptosis or enhance proliferation of a cancer cell (35).
The role of the G2 arrest in stem cell quiescence appears functionally and mechanistically different from G2 arrest in differentiated cells. Whether the G2 arrest takes place in embryonic stem cells (as in muscle zebrafish) or in adult stem cells (as in the abovementioned cases), it is associated with robust regeneration capacity. Moreover, in all of these cases, the G2 arrest is reversible and released when regeneration must be activated. Does G2 arrest provide advantages that ultimately promote robust regeneration? And if this is the case, which regulatory factors are implicated? The genome of cells in G2 is replicated and these cells are poised to undergo mitosis when the proper signal is provided. Perhaps having already undergone the sensitive phases of DNA replication and correction of DNA damage positions stem cells in a favorable condition for efficient proliferation and self-renewal when the embargo is lifted. Perhaps re-entry into the cell cycle is easier, since the G2/M checkpoint is not as robust as the G1/S checkpoint, at least in the context of DNA damage (36). Alternatively, cells in G2 may have characteristics that are relevant to the next steps; as an example, cells arrested in mitosis tend to adhere less well to the neighboring cells than cells in other phases of the cell cycle. It is also possible that G2 arrest is advantageous for short periods that are followed by rapid and numerous cycles of proliferation and self-renewal. This situation contrasts with satellite cells that display long periods of quiescence.
Conclusions
The reasons of clonal drift and progressive loss of stem cell heterogeneity in adult and embryonic organ growth remain to be elucidated. This process appears to be dependent on the type of stem cell, rather than the type of organism, at least with the limited examples available to date. Heterogeneity is perhaps a requirement for stem cells that undergo extensive periods of quiescence, in order to maintain specialized subpopulations to face a variety of conditions when regeneration is activated. The establishment of stem cell diversity, perhaps favored by asymmetric division that generates different daughter cells (10,37), its possible refinement during inactive phases, as well as the maintenance of this diversity during time appear to be necessary conditions for this type of regeneration. Conversely during clonal drift, the success of expansion (although of a few stem cells) prevails over the maintenance of diverse stem cell pools, and this suggests a process where stem cell heterogeneity is perhaps not functional to the regeneration process. Loss of stem cell heterogeneity seems to favor conditions of almost continuous expansion interspaced by relatively short periods of quiescence (embryonic growth, repetitive muscle injury) and also stem cells localized in restricted structures (e.g., intestinal stem cells). In these conditions, cells normally undergo symmetric divisions, and the original stem cell diversity lost by apparently random events. These salient features of this randomness are beginning to be identified, like the Meox1 protein in the zebrafish myotome, although we cannot presently exclude the possibility that determinant factors might concomitantly direct stem cell fates.
Muscle stem cells in the zebrafish embryo are maintained as such by blockage in the G2 phase of the cell cycle, a condition that has been reported in other cases, and this event appears to be linked to robust regeneration potential. Conversely, arrest in G2 phase in differentiated cells is essentially linked to ensure genome stability and its outcome is at the crossroad of cell proliferation, apoptosis, cellular senescence, and clonal expansion of cancer cells. The salient properties of stem cells, namely self-renewal and differentiation, may help us to understanding in more detail the impact of G2 arrest in cells with a highly proliferative potential.
Acknowledgements
M Ricchetti thanks Japan Aerospace Exploration Agency (JAXA) "Living in Space" program for support and Dr. Atsuko Sehara, Kyoto University, Japan, for helpful discussion.
This work was supported by Association Française contre les Myopathies (AFM, PhD grant to HH Sutcu).
Footnote
Conflicts of Interest: The authors have no conflicts of interest to declare.
References
- Tajbakhsh S. Skeletal muscle stem cells in developmental versus regenerative myogenesis. J Intern Med 2009;266:372-89. [Crossref] [PubMed]
- Motohashi N, Asakura A. Muscle satellite cell heterogeneity and self-renewal. Front Cell Dev Biol 2014;2:1. [Crossref] [PubMed]
- Tierney MT, Sacco A. Satellite Cell Heterogeneity in Skeletal Muscle Homeostasis. Trends Cell Biol 2016;26:434-44. [Crossref] [PubMed]
- Picard CA, Marcelle C. Two distinct muscle progenitor populations coexist throughout amniote development. Dev Biol 2013;373:141-8. [Crossref] [PubMed]
- Harel I, Nathan E, Tirosh-Finkel L, et al. Distinct origins and genetic programs of head muscle satellite cells. Dev Cell 2009;16:822-32. [Crossref] [PubMed]
- Sambasivan R, Gayraud-Morel B, Dumas G, et al. Distinct regulatory cascades govern extraocular and pharyngeal arch muscle progenitor cell fates. Dev Cell 2009;16:810-21. [Crossref] [PubMed]
- Kassar-Duchossoy L, Giacone E, Gayraud-Morel B, et al. Pax3/Pax7 mark a novel population of primitive myogenic cells during development. Genes Dev 2005;19:1426-31. [Crossref] [PubMed]
- Ono Y, Boldrin L, Knopp P, et al. Muscle satellite cells are a functionally heterogeneous population in both somite-derived and branchiomeric muscles. Dev Biol 2010;337:29-41. [Crossref] [PubMed]
- Collins CA, Partridge TA. Self-renewal of the adult skeletal muscle satellite cell. Cell Cycle 2005;4:1338-41. [Crossref] [PubMed]
- Rocheteau P, Gayraud-Morel B, Siegl-Cachedenier I, et al. A subpopulation of adult skeletal muscle stem cells retains all template DNA strands after cell division. Cell 2012;148:112-25. [Crossref] [PubMed]
- Chakkalakal JV, Jones KM, Basson MA, et al. The aged niche disrupts muscle stem cell quiescence. Nature 2012;490:355-60. [Crossref] [PubMed]
- Latil M, Rocheteau P, Chatre L, et al. Skeletal muscle stem cells adopt a dormant cell state post mortem and retain regenerative capacity. Nat Commun 2012;3:903. [Crossref] [PubMed]
- Sousa-Victor P, Gutarra S, Garcia-Prat L, et al. Geriatric muscle stem cells switch reversible quiescence into senescence. Nature 2014;506:316-21. [Crossref] [PubMed]
- Conboy IM, Conboy MJ, Wagers AJ, et al. Rejuvenation of aged progenitor cells by exposure to a young systemic environment. Nature 2005;433:760-4. [Crossref] [PubMed]
- Garcia-Prat L, Martinez-Vicente M, Perdiguero E, et al. Autophagy maintains stemness by preventing senescence. Nature 2016;529:37-42. [Crossref] [PubMed]
- Ricchetti M. How stem cells manage to escape senescence and ageing - while they can: A recent study reveals that autophagy is responsible for senescence-dependent loss of regenerative potential of muscle stem cells during ageing. Bioessays 2016;38:857-62. [Crossref] [PubMed]
- Snippert HJ, van der Flier LG, Sato T, et al. Intestinal crypt homeostasis results from neutral competition between symmetrically dividing Lgr5 stem cells. Cell 2010;143:134-44. [Crossref] [PubMed]
- Lopez-Garcia C, Klein AM, Simons BD, et al. Intestinal stem cell replacement follows a pattern of neutral drift. Science 2010;330:822-5. [Crossref] [PubMed]
- Aghaallaei N, Gruhl F, Schaefer CQ, et al. Identification, visualization and clonal analysis of intestinal stem cells in fish. Development 2016;143:3470-80. [Crossref] [PubMed]
- Snippert HJ, Schepers AG, van Es JH, et al. Biased competition between Lgr5 intestinal stem cells driven by oncogenic mutation induces clonal expansion. EMBO Rep 2014;15:62-9. [Crossref] [PubMed]
- Kronen MR, Schoenfelder KP, Klein AM, et al. Basolateral junction proteins regulate competition for the follicle stem cell niche in the Drosophila ovary. PLoS One 2014;9:e101085. [Crossref] [PubMed]
- Tierney MT, Stec MJ, Rulands S, et al. Muscle Stem Cells Exhibit Distinct Clonal Dynamics in Response to Tissue Repair and Homeostatic Aging. Cell Stem Cell 2018;22:119-27.e3. [Crossref] [PubMed]
- Martincorena I, Roshan A, Gerstung M, et al. Tumor evolution. High burden and pervasive positive selection of somatic mutations in normal human skin. Science 2015;348:880-6. [Crossref] [PubMed]
- Jaiswal S, Fontanillas P, Flannick J, et al. Age-related clonal hematopoiesis associated with adverse outcomes. N Engl J Med 2014;371:2488-98. [Crossref] [PubMed]
- Gupta V, Poss KD. Clonally dominant cardiomyocytes direct heart morphogenesis. Nature 2012;484:479-84. [Crossref] [PubMed]
- McKenna A, Findlay GM, Gagnon JA, et al. Whole-organism lineage tracing by combinatorial and cumulative genome editing. Science 2016;353:aaf7907. [Crossref] [PubMed]
- Nguyen PD, Gurevich DB, Sonntag C, et al. Muscle Stem Cells Undergo Extensive Clonal Drift during Tissue Growth via Meox1-Mediated Induction of G2 Cell-Cycle Arrest. Cell Stem Cell 2017;21:107-19.e6. [Crossref] [PubMed]
- Mankoo BS, Skuntz S, Harrigan I, et al. The concerted action of Meox homeobox genes is required upstream of genetic pathways essential for the formation, patterning and differentiation of somites. Development 2003;130:4655-64. [Crossref] [PubMed]
- Petropoulos H, Gianakopoulos PJ, Ridgeway AG, et al. Disruption of Meox or Gli activity ablates skeletal myogenesis in P19 cells. J Biol Chem 2004;279:23874-81. [Crossref] [PubMed]
- Douville JM, Cheung DY, Herbert KL, et al. Mechanisms of MEOX1 and MEOX2 regulation of the cyclin dependent kinase inhibitors p21 and p16 in vascular endothelial cells. PLoS One 2011;6:e29099. [Crossref] [PubMed]
- Munoz-Espin D, Serrano M. Cellular senescence: from physiology to pathology. Nat Rev Mol Cell Biol 2014;15:482-96. [Crossref] [PubMed]
- Rumman M, Dhawan J, Kassem M. Concise Review: Quiescence in Adult Stem Cells: Biological Significance and Relevance to Tissue Regeneration. Stem Cells 2015;33:2903-12. [Crossref] [PubMed]
- Buzgariu W, Crescenzi M, Galliot B. Robust G2 pausing of adult stem cells in Hydra. Differentiation 2014;87:83-99. [Crossref] [PubMed]
- Heber-Katz E, Zhang Y, Bedelbaeva K, et al. Cell cycle regulation and regeneration. Curr Top Microbiol Immunol 2013;367:253-76. [Crossref] [PubMed]
- Sperka T, Wang J, Rudolph KL. DNA damage checkpoints in stem cells, ageing and cancer. Nat Rev Mol Cell Biol 2012;13:579-90. [Crossref] [PubMed]
- Lobrich M, Jeggo PA. The impact of a negligent G2/M checkpoint on genomic instability and cancer induction. Nat Rev Cancer 2007;7:861-9. [Crossref] [PubMed]
- Yennek S, Burute M, Thery M, et al. Cell adhesion geometry regulates non-random DNA segregation and asymmetric cell fates in mouse skeletal muscle stem cells. Cell Rep 2014;7:961-70. [Crossref] [PubMed]
Cite this article as: Sutcu HH, Ricchetti M. Loss of heterogeneity, quiescence, and differentiation in muscle stem cells. Stem Cell Investig 2018;5:9.