Aberrant RNA splicing and mutations in spliceosome complex in acute myeloid leukemia
Introduction
Hematological malignancies are a group of malignant diseases originating from blood, bone marrow cells or lymph, including leukemia [myeloid originated: chronic myeloid leukemia (CML), acute myeloid leukemia (AML); lymphoid originated: chronic lymphoblastic leukemia (CLL) and acute lymphoblastic leukemia (ALL)], plasma multiple myeloma (MM) (plasma cell originated) and lymphoma [non-Hodgkin lymphoma (NHL) and Hodgkin lymphoma (HL)] (1). Depending on the type of leukemia and the age of the patient at diagnosis, the prognosis of leukemia patients differs significantly. However, in general, leukemia is the 5th and 6th most common cancer death in men and women, respectively (Facts and Statistics 2015, Leukemia and Lymphoma Society). AML remains one of the worst clinically devastating diseases. The 5-year overall survival rate is about 20% in adult AML patients (2,3). These poor outcomes highlight the unmet need for a better understanding of leukemogensis and novel, targeted therapies to replace chemotherapy, which has not been changed for more than four decades (4-7).
Leukemia stem cell (LSC) was described as source of origin and progression of AML in more than 10 years ago (8). Accumulating evidence supports the fact that these LSC populations acquire self-renewal function and sustain the disease (9,10). They are rare, but functionally and phenotypically different form bulk of blast cells (11,12). AML LSCs are generally insensitive to the conventional chemotherapy, instead, they are more enriched after chemotherapy (13,14). Physically, LSC populations reside in the bone marrow microenvironment and are poised to propagate, leading to the therapy failure and disease relapse (15-18). Taken together, these discoveries indicate that the LSC is the culprit for the dismal prognosis of AML and selectively targeting LSC could be a promising strategy for AML treatment (19-23).
The advent of next generation sequencing (NGS) technologies has revolutionized the field of genomics, enabling us to gain a deep and broad understanding of human diseases on a whole genome level, including cancers (24-26). Recent studies using NGS platform have revealed substantially frequency of recurrent somatic points and copy number changes in genes associated with spliceosome in leukemias and myelodysplastic syndromes (MDS), which are chronic myeloid neoplasms, often progressing to AML (27-30). This review will first describe the complex and general function of RNA spliceosome. We will outline various mis-spliced mRNA in AML and their clinical significance, followed by summary of mutations in spliceosome and related factors, particularly its role in AML LSC. Finally, we will discuss the promises and challenges in an attempt to exploit spliceosomal machinery therapeutically.
The spliceosomal machinery and RNA splicing regulation
In mammalian cells, genes are transcribed as messenger RNA precursors (pre-mRNAs), containing introns, which are intervening sequences, noncoding regions (31). RNA splicing, the process from nuclear pre-mRNA into mature mRNA where introns are excised and the exons (coding regions) are joined together is mediated by a large complex, called spliceosome or spliceosomal machinery (32,33). In human genome, 60% of the mature mRNAs are spliced by alternative splicing in which pre-mRNAs can be spliced in more than one way (34,35). Alternative splicing tremendously increases the diversity of human transcriptome, resulting in translation of more complex proteome in human. Alternative splicing also acts as a form of gene regulation because dominant negative protein translated from alternative spliced mRNA can inhibit its wild type protein. Thus, RNA splicing ultimately influences assorted cellular functions, tissue specificity, and developmental states of human.
The spliceosome is a large molecular machinery, consisting of five small nuclear ribonucleoproteins (snRNPs) (RNA-protein complex), U1, U2, U4, U5 and U6, as well as about 200 associated protein factors, such as serine and arginine-rich proteins (SR proteins) and heterogeneous nuclear ribonucleoprotein (hnRNP) family (36,37). The boundaries between introns and exons of the pre-mRNAs are distinguished by specific nucleotide sequences. The 5' splice site at the 5' (left) end of the intron includes almost an invariant sequence GU, while the splice acceptor site at the 3' splice site (right) end of the intron includes an consensus sequence AG (GU-AG rule) (38). In general, the RNA splicing process involves three steps, recognizing the appropriate splice sites, bringing those sites together, and catalyzing the splicing reactions. U1 snRNP initiates splicing by recognizing the 5' splice site and binding it through an RNA-RNA base pairing reaction (canonical Watson-Crick or wobble base pairs), resulting in the assembly of the commitment (E) complex. U1 snRNP plays an important role in the binding of U2 snRNP to the branch point region of the intron. Subunit of U2 snRNP, U2 auxiliary factors (U2AFs) bind to polypyrimidine tract region and the other to the intron’s 3' splice site-AG dinucleotide. The association of both of the U1 snRNP and U2 snRNP assembles the pre-spliceosome (complex A). U4, U5 and U6 form the tri-snRNP complex. When pre-spliceosome recruits this tri-snRNP complex, it converts the A complex into B complex, which comprises all the necessary splicing components and poises to catalyze. The B complex passes through a series of RNA-RNA rearrangements and transform into C complex, in which U2 and U6 are brought together to create the catalytic active site. In human, 99% of the introns is cleaved by this way (major spliceosome). An alternative splicing pathway uses the minor spliceosome which contains U12 and another set of snRNPs in a similar fashion. For the purpose of this review, here we only briefly outline the spliceosome formation and splicing process. We recommend a number of comprehensive and elegant reviews for a detailed coverage of this topic (39-42).
Abnormally spliced (AS) mRNAs in AML
Before the advent of NGS technology, abnormal RNA splicing of certain oncogenes, tumor suppressor gene and epigenetic regulators had been noticed in AML, although in an unsystematic fashion. Here we have concisely summary some of these main findings.
Myc gene belongs to Myc family of transcription factors, which comprises C-Myc, N-Myc and L-Myc genes. Myc gene is a prominent oncogene and has been implicated in transformation of many types of cancers, including leukemia (43). A unique pattern of L-Myc mRNA processing with 40% of them lacking exon III and intron I has been revealed in AML (44). Importantly, L-Myc expression is very low in adult bone marrow and in fetal spleen and thymus. However, whether this splicing variant functionally contributes to leukemogenicity has not been defined. In contrast, two isoforms of Kit transcripts created through the alternate use of 5' splice donor sites (alternative splicing) are detected in AML (45), but further analysis showed no apparent association with pathology of AML, indicating naturally occurring changes in splicing mechanisms as stem cells differentiate (46). An alternatively spliced IL-6R mRNA, encoding soluble IL-6R (sIL-6R) expressed in 64% of the primary blast cells of AML patients and all AML cell lines tested, supporting the notion of alternative splicing as a mechanism of sIL-6R production in AML (47).
PTPN6 is a 68 kDa SH2 domain-containing tyrosine phosphatase. PTPN6 regulates hematopoietic cell development, proliferation and receptor-mediated mitogenic signaling pathways (48). A novel PTPN6 mRNA species, derived from aberrant splicing within the N-SH2 domain leading to retention of intron 3 has been discovered in CD34(+)/CD117(+) blasts from AML patients (49). The level of the aberrant intron-retaining splice variant, is lower in CD117(+)-AML bone marrow mononuclear cells at remission than at diagnosis, suggesting the involvement of post-transcriptional PTPN6 processing in leukemogenesis (49).
Survivin is a member of the inhibitors of apoptosis protein family, playing important roles in cell proliferation and survival (50). Survivin is highly expressed in CD34+/CD38− leukemia progenitor cells and associated poor prognosis and drug resistance in AML patients (51-54). In addition, surviving has been shown to selectively modulate genes the epidermal growth factor receptor signaling pathway in AML LSCs (55). It has been long recognized that alternative splicing of its pre-mRNA generates four different mRNAs: survivin, survivin-2B, survivin-ΔEx3 and survivin-3B (56,57). In AML cells, survivin is the predominant transcript variant, whereas significantly survivin-2B and survivin-DeltaEx3 express at lower level (58). Expression patterns of survivin variants are associated with clinical outcome. For example, expression of survivin-3B is detected in AML cell lines and may associate with G2/M phase of cell cycle (59). Low expression of survivin-2B correlated with a better overall survival and event-free-survival, whereas high survivin-DeltaEx3 expression was associated with a shorter overall survival (58). However, these splice variants don’t correlate with FAB subtypes, immunophenotype or cytogenetic risk groups (58). These studies support the conclusion that certain survivin splice variants have prognostic values and could be implicated in the leukemogensis of AML.
Hoxa9 belongs to a family of homeodomain containing transcription factors (60). Hox family regulates genes which control the anterior-posterior body plan and assign tissue fate in human (61). Dysregulation of Hoxa9 has been found in more than 50% of AML patients and highly predicts worse survival (62). Research data indicate splicing play a central role in Hox gene mediated leukemogenesis as a full-length Hoxa9 engineered to prevent natural splicing significantly reduced in vivo leukemogenicity (62).
Oncogene Wilms’ tumor gene 1 (WT1) is a zinc-finger motif containing transcription factor with a proline/glutamine-rich DNA-binding domain. WT1 has been a target for immunotherapy and biomarker commonly used in monitoring of minimal residual disease (MRD) in AML patients. A large assortment of isoforms of WT1 transcripts as many as 36 has been identified. Among them, +5/+KTS are the predominant variants at diagnoses, but their ratio vary between diagnoses (63-66). Increased ratio of the +5/−KTS is associated with aggressive and/or resistant characteristics in FAB subtype M3 and secondary AML (sAML) (63). Together, these data suggest the ratio of certain WT1 isoforms might be crucial for transformation of AML or relapse (66).
The FMS-like tyrosine kinase 3 (FLT3) is a class III RTK family, sharing structural similarity with platelet-derived growth factors (PDGFRs), the colony-stimulating factor 1 receptor (CSF1-R) and steel factor receptor (KIT) (67,68). FLT3 mutations are identified in about one-third of adult (AML) (69,70). FLT3 mutations induce constitutive activation of phosphoinositide 3-kinase (PI3K)-AKT, RAS-MEK-mitogen-activated protein kinase (MAPK), and signal transducers and activators of transcription (STAT) five pathways, leading uncontrolled cell proliferation, blockage of differentiation and cell survival (52). Thus, FLT3 mutations play a central role in leukemogenesis (71-73). Surprisingly, FLT3, together with NOTCH2 has been identified as the most commonly mis-spliced genes in more than 70% of AML patients (74). The splice variants of NOTCH2 and FLT3 are produced through complete or partial exon skipping and utilization of cryptic splice sites (75). NOTCH2 and FLT3 aberrant splicing is observed in more than 70% of AML cases at diagnosis and their expressions decrease at remission. NOTCH-2Va and FLT3-Va transcripts are detected in a significant number of AML patients and high level of NOTCH-2Va predicts worse outcome independent of other known clinical indicators (75).
Taken together, accumulating evidence clearly demonstrate that mis-splicing of certain genes is a common characteristic of AML and some of these mis-spliced mRNAs could translate into proteins with altered function which contribute to leukemogenesis (Table 1). Notably, there is great interest in identification of splice variants of these genes as disease markers for both diagnosis and stratification and targets for novel therapeutics of leukemia patients.
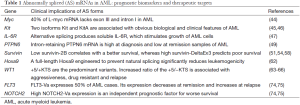
Full table
Aberrant RNA splicing and drug resistance in AML
Although initial response to chemotherapy, most of AML patients will develop resistance (76). The appearance of resistance poises a major therapeutic challenge in the treatment of AML and is the primer cause of mortality (77). The phenomenon when an untreated patient does not respond to chemotherapy is termed as primary resistance or intrinsic resistance. Secondary resistance (or acquired resistance) is almost unavoidable when chemotherapy is used for a long period. Alternative RNA splicing also contributes to drug resistance in AML. Cytarabine (Ara-C) is one of the core drugs in the combination chemotherapy against AML (78). Low expression or activity of deoxycytidine kinase (dCK) is responsible for the in vitro cellular resistance to Ara-C in AML cells. Only wild-type mRNA of dCK is amplified from healthy control samples, while splicing variants translating inactive dCK protein resulting from exon skipping are detected in 7 out of 12 purified AML specimen from resistant patients (79). Further work indicates that the alternatively spliced dCK forms render the AML cells to evade to Ara-C attack when there is no wide type dCK (80). On the other side of the coin, exon-array analysis has been performed in isogenic sensitive and (secondary) resistant AML cell lines to Ara-C, doxorubicin (Dox) and hypomethylating agent, azacitidine (Aza) and produced novel insight of alternative exon usages (AEUs) globally (81). Significant alternations were identified in near 1,000 AEU events in a few thousand genes on average between these sensitive and resistant cell lines. GO analysis uncovers five common functional pathways that are shared with resistance to Ara-C, Dox or Aza, including T cell receptor signaling pathway, focal adhesion, axon guidance, regulation of actin cytoskeleton, and ECM-receptor interaction. Taken together, aberrant RNA splicing could act as both cause and consequence of drug resistance in AML, suggesting that targeting RNA splicing process might be a novel approach to overcome resistance in anti-AML therapy (82).
Aberrant RNA splicing and genetic alternations of spliceosome or splicing factors in AML
NGS analysis of MDS in 2011 unexpectedly discovered recurrent somatic mutation of splicing factor 3b subunit 1 (SF3B1) in 20% of cases (27,83). SF3B1 is a component of the U2 snRNP complex and binds pre-mRNA upstream of the intron’s branch site (39). Following this initial finding, a number of studies aiming to categorize genetic alternations in spliceosome and related factors in AML have been performed. Although the frequencies of mutations in genes involving splicing process is relatively low in AML as compared to MDS, these abnormalities influence the overall survival rate and contribute to pathogenesis of AML. One recent study collecting more than 1,500 samples provided an unrivalled understanding of how different driver mutations cooperate and lead to AML (84). In this elegant study, a new molecular subgroup of AML with mutations in genes encoding chromatin, RNA-splicing regulators, or both is defined and occurs in 18% of patients. The common mutated splicing modulators encompass SRSF2, SF3B1, U2AF1 and ZRSR2. SRSF2 is a member of the serine (S) and arginine (R) rich family of pre-mRNA splicing factors, consisting of an RNA recognition motif (RRM) and an RS domain (84). SRSF2 protein regulates diverse RNA-related processes, enhancing the U1 snRNP complex binding to the 5' splice site of pre-mRNA, U2 snRNP binding at the branch point, and mRNA stabilization. Clinically, AML patients carrying spliceosome-chromatin mutations has poor prognosis. Furthermore, co-occurrence of TP53 mutations in this group of patients makes their prognosis even worse. In practice, these patients should be the candidates for clinical trials of investigational agents or bone marrow transplant (BMT) (84). Recurrent somatic mutations in U2AF1 (also known as U2AF35) have been identified in 3–4% of AML cases. There were 369 splicing alterations significantly associated with U2AF1 mutation (84).
DEAD-box polypeptide 41 (DDX41) (also known as ABS) is an evolutionarily conserved germ cell marker in a wide range of animals. DDX41 belongs to an RNA helicase family, characterized by the conserved motif Asp-Glu-Ala-Asp (DEAD) (85). DDX4 plays an important role in RNA spliceosome assembly, and many other processes including translation initiation, pre-ribosomal RNA processing, small RNA biogenesis and chromosome condensation (85). DDX41 mutations have been identified in about 3% in inherited hematologic malignancies (HM) (86). MDS and AML are the most common malignancies with lower-age onset (87). Defection of DDX41 sizably impair pre-mRNA splicing on the evidence of more avid exon skipping and more exon retention in 61 and 95 genes, respectively, observed in mutant cases than controls (87). Recently, resurgence of AML in donor cells in a patient after allogeneic BMT in a family with a germline DDX41 mutation (88). Taken together, these studies strongly support DDX41 as a tumor suppressor gene and defective DDX41 contributes to AML development.
PRE-mRNA processing factor 8 (PRPF8) shares high identity (61%) in amino acid sequences from yeast to human and plays a central position in the catalytic core of the spliceosome (89). Keightley and colleagues first reported that mutant PRPF8 carrying an early premature STOP codon instigate aberrantly spliced transcripts retaining both U2- and U12-type introns in zebrafish model (90). These authors also observed that myeloid differentiation was impaired within early haematopoiesis (90). It is worthy of pointing out that blocked differentiation of myeloid cells is one of the characteristic feature of AML. Combing these evidences, we could imply that PRPF8 is required for haematopoietic development and defective PRPF8 could be conducive to myeloid malignancies. Indeed, Kurtovic-Kozaric and coworkers identified either recurrent somatic PRPF8 mutations or hemizygous deletions in 3.3% (15 out of 447 cases) and 5.3% (24 out of 450 cases) of myeloid neoplasms, respectively (91). Notably, 50% of PRPF8 mutant and deletion of chromosome 17p cases were found in AML. Survival analysis confirmed that PRPF8 was associated with poor prognosis (91).
Splicing factor proline and glutamine rich (SFPQ) is one of the three member Drosophila behavior/human splicing (DBHS) family in human (92). The DBHS family shares highly conserved tandem N-terminal RRMs, a NonA/paraspeckle domain (NOPS) and a C-terminal coiled-coil (93). SFPQ binds wide range of nucleic acids and can be found in the pre-assembled spliceosome complex. However, SFPQ is not an essential member of spliceosome, but rather act as a molecular scaffold and involve co-translational and alternative splicing (93). Mutations in SFPQ and in the nonclassic regulators of mRNA processing CTCF and RAD21 have been discovered in 10% of AML patients in a mutually exclusive manner (94). However, the clinical importance of this mutation has not been confirmed.
In addition to mutations occurring the spliceosome and related factors, the expression levels of some splicing factor SR protein family are also downregulated in AML samples relative to healthy controls (95). Moreover, abnormal caspase-8 pre-mRNA splicing is observed only in AML patients, has significant correlation with several splicing factors (95). These data imply that aberrant expression of splicing factors may potentially rewire apoptosis pathway in AML.
Distinct deregulation of RNA splicing in sAML and LSC
sAML or therapy-related AML refers to this disease arises from patients who previously received chemotherapy and/or radiation therapy (96). These patients often have featured genetic changes, including complex karyotype, chromosome 11q abnormalities (MLL gene arrangements), and monosomy of chromosome 5 or 7, p53 mutations (97). Clinically, patients with sAML often are resistant to chemotherapy and have extremely dismal outcome with average survival of 7 months in 40% of patients (97). Thus, a better apprehension of the molecular pathology of sAML and finding novel treatment option are the areas of unmet need.
A recent study by Crews et al. sparks substantial excitement surrounding the pivotal role of spliceosome in sAML and the therapeutic potential of targeting LSCs in this subtype of AML often unresponsive to current therapy (98). In this report, whole-transcriptome sequencing was performed from purified progenitors isolated from sAML, de novo AML and MDS patients. Although mutation in U2 splicing factor SF3B1 was only identified in one sAML sample, increased expression of wild-type SF3B1 was confirmed in a subset of sAML LSCs (98). A splice isoform signature of sAML LSCs was created, which was represented by several alternatively spliced signal transduction and cell adhesion gene products. Pro-survival long isoforms of BCL2 family was elevated in sAML compared to young and aged normal hematopoietic progenitor cells (HPCs), indicating existence of pro-survival splice isoform switching in LSC transformation (98). Over the past 10 years, a list of natural products, including FD-895, pladienolide B, herboxidiene, and spliceostatin A, have been identified as spliceosome modulators (99). They have been shown to have anti-cancer effect in vitro and in vivo models. However, these compounds demonstrate poor metabolic stability and short half-lives in vivo, excluding them from entering clinical evaluation (99,100). 17S-FD-895, an analog of FD-895, was synthesized through the combination of total synthesis and synthetic methods, demonstrating improved stability and on-target effect (101). This new spliceosome targeting compound was evaluated in different sAML models and showed potent efficacy in inhibition of AML LSC and disruption of AML maintenance in vitro and in mouse xenograft models (98). Importantly, 17S-FD-895 minimal impacted normal BM HSPCs. To validate its on-target effect, comparative RNA-seq analysis revealed that the splice isoform signature of sAML LSCs has been reversed to a normal BM transcriptome profile in treated mice (11). In sum, these evidence underscore that eradication of LSC by targeting spliceosome could represent a novel therapeutic strategy for sAML and further support the development of 17S-FD-895 in clinic.
Outlook
With the aid of rapid progress in NGS technology and bioinformatics, novel recurrent mutations and expression level changes in the spliceosome and splicing regulating factors have been uncovered. A flurry of studies characterizing spliceosomal abnormalities in various hematological malignancies and solid tumors have been published since 2011. Also, the promise of the spliceosome as novel anticancer target has drawn substantial funding and focus from both academic and pharma-industrial. We would anticipate a line of such novel therapeutic agents will be tested in clinical trials in near future. However, cautions must be highlighted as we still don’t understand well how these aberrant RNA splicing exactly contribute the development of cancers. Importantly, as proper splicing is required for normal hematopoiesis, the potential side effect of manipulating spliceosome has not been addressed in human in a long term.
In addition to the improvement of current compounds in terms of better pharmacokinetics (PK), distribution and pharmacodynamics (PD) in human, substantial efforts have been devoted into additional small molecular inhibitor library screening to discover new class of spliceosome inhibitors with much favorable therapeutic index. Furthermore, another therapeutic approach by gene-silencing molecules with antisense oligonucleotide derivatives or small interfering RNA (siRNA) for targeting aberrant splicing activity has attracted considerable attention. As compared to small molecular inhibitors, oligonucleotide-based therapeutics offer the promise of precise targeting any mutant splicing factors without impeding any gene sharing sequencing similarity. Although currently no case of such gene-silencing molecules has been approved by government regulators, the difficulty of, with the aid of rapid progress in in vivo delivery field, we would anticipate a line of such oligonucleotide-based novel therapeutic agents will be tested in anti-AML trials in near future.
Acknowledgements
Due to space limit, the authors apologize to those whose important works in this field could not be discussed.
Funding: This research is supported by the National Research Foundation Singapore and the Singapore Ministry of Education under its Research Centres of Excellence initiative and NMRC Clinician-Scientist IRG Grant CNIG11nov38 (J Zhou). WJ Chng is also supported by NMRC Clinician Scientist Investigator award.
Footnote
Conflicts of Interest: The authors have no conflicts of interest to declare.
References
- Harris NL, Jaffe ES, Diebold J, et al. The World Health Organization classification of hematological malignancies report of the Clinical Advisory Committee Meeting, Airlie House, Virginia, November 1997. Mod Pathol 2000;13:193-207. [Crossref] [PubMed]
- Dores GM, Devesa SS, Curtis RE, et al. Acute leukemia incidence and patient survival among children and adults in the United States, 2001-2007. Blood 2012;119:34-43. [Crossref] [PubMed]
- Shipley JL, Butera JN. Acute myelogenous leukemia. Exp Hematol 2009;37:649-58. [Crossref] [PubMed]
- Lynch RC, Medeiros BC. Chemotherapy options for previously untreated acute myeloid leukemia. Expert Opin Pharmacother 2015;16:2149-62. [Crossref] [PubMed]
- Zhou J, Ching YQ, Chng WJ. Aberrant nuclear factor-kappa B activity in acute myeloid leukemia: from molecular pathogenesis to therapeutic target. Oncotarget 2015;6:5490-500. [Crossref] [PubMed]
- Ng AP, Chng WJ, Khan M. Curcumin sensitizes acute promyelocytic leukemia cells to unfolded protein response-induced apoptosis by blocking the loss of misfolded N-CoR protein. Mol Cancer Res 2011;9:878-88. [Crossref] [PubMed]
- Abdollahi P, Vandsemb EN, Hjort MA, et al. Src Family Kinases Are Regulated in Multiple Myeloma Cells by Phosphatase of Regenerating Liver-3. Mol Cancer Res 2017;15:69-77. [Crossref] [PubMed]
- Bonnet D, Dick JE. Human acute myeloid leukemia is organized as a hierarchy that originates from a primitive hematopoietic cell. Nat Med 1997;3:730-7. [Crossref] [PubMed]
- Zhou J, Ng SB, Chng WJ. LIN28/LIN28B: an emerging oncogenic driver in cancer stem cells. Int J Biochem Cell Biol 2013;45:973-8. [Crossref] [PubMed]
- Muench DE, Grimes HL. Transcriptional Control of Stem and Progenitor Potential. Curr Stem Cell Rep 2015;1:139-50. [Crossref] [PubMed]
- Heidel FH, Mar BG, Armstrong SA. Self-renewal related signaling in myeloid leukemia stem cells. Int J Hematol 2011;94:109-17. [Crossref] [PubMed]
- Koh CP, Ng CE, Nah GS, et al. Hematopoietic stem cell enhancer: a powerful tool in stem cell biology. Histol Histopathol 2015;30:661-72. [PubMed]
- Ossenkoppele G, Schuurhuis GJ. MRD in AML: does it already guide therapy decision-making? Hematology Am Soc Hematol Educ Program 2016;2016:356-65. [Crossref] [PubMed]
- Lin S, Zhao R, Xiao Y, et al. Mechanisms determining the fate of hematopoietic stem cells. Stem Cell Investig 2015;2:10. [PubMed]
- Zhou J, Mauerer K, Farina L, et al. The role of the tumor microenvironment in hematological malignancies and implication for therapy. Front Biosci 2005;10:1581-96. [Crossref] [PubMed]
- Ishikawa F, Yoshida S, Saito Y, et al. Chemotherapy-resistant human AML stem cells home to and engraft within the bone-marrow endosteal region. Nat Biotechnol 2007;25:1315-21. [Crossref] [PubMed]
- Stahl M, Kim TK, Zeidan AM. Update on acute myeloid leukemia stem cells: New discoveries and therapeutic opportunities. World J Stem Cells 2016;8:316-31. [Crossref] [PubMed]
- Kang B, Sun XH. Regulation of cancer stem cells by RING finger ubiquitin ligases. Stem Cell Investig 2014;1:5. [PubMed]
- Felipe Rico J, Hassane DC, Guzman ML. Acute myelogenous leukemia stem cells: from Bench to Bedside. Cancer Lett 2013;338:4-9. [Crossref] [PubMed]
- Majeti R, Chao MP, Alizadeh AA, et al. CD47 is an adverse prognostic factor and therapeutic antibody target on human acute myeloid leukemia stem cells. Cell 2009;138:286-99. [Crossref] [PubMed]
- Ye M, Zhang H, Yang H, et al. Hematopoietic Differentiation Is Required for Initiation of Acute Myeloid Leukemia. Cell Stem Cell 2015;17:611-23. [Crossref] [PubMed]
- Kikushige Y, Akashi K. TIM-3 as a therapeutic target for malignant stem cells in acute myelogenous leukemia. Ann N Y Acad Sci 2012;1266:118-23. [Crossref] [PubMed]
- Askmyr M, Agerstam H, Hansen N, et al. Selective killing of candidate AML stem cells by antibody targeting of IL1RAP. Blood 2013;121:3709-13. [Crossref] [PubMed]
- Koboldt DC, Steinberg KM, Larson DE, et al. The next-generation sequencing revolution and its impact on genomics. Cell 2013;155:27-38. [Crossref] [PubMed]
- Welch JS, Link DC. Genomics of AML: clinical applications of next-generation sequencing. Hematology Am Soc Hematol Educ Program 2011;2011:30-5. [Crossref] [PubMed]
- Das S, Krainer AR. Emerging functions of SRSF1, splicing factor and oncoprotein, in RNA metabolism and cancer. Mol Cancer Res 2014;12:1195-204. [Crossref] [PubMed]
- Papaemmanuil E, Cazzola M, Boultwood J, et al. Somatic SF3B1 mutation in myelodysplasia with ring sideroblasts. N Engl J Med 2011;365:1384-95. [Crossref] [PubMed]
- Graubert TA, Shen D, Ding L, et al. Recurrent mutations in the U2AF1 splicing factor in myelodysplastic syndromes. Nat Genet 2011;44:53-7. [Crossref] [PubMed]
- Inoue D, Bradley RK, Abdel-Wahab O. Spliceosomal gene mutations in myelodysplasia: molecular links to clonal abnormalities of hematopoiesis. Genes Dev 2016;30:989-1001. [Crossref] [PubMed]
- Larsson CA, Cote G, Quintas-Cardama A. The changing mutational landscape of acute myeloid leukemia and myelodysplastic syndrome. Mol Cancer Res 2013;11:815-27. [Crossref] [PubMed]
- Lee Y, Rio DC. Mechanisms and Regulation of Alternative Pre-mRNA Splicing. Annu Rev Biochem 2015;84:291-323. [Crossref] [PubMed]
- Irimia M, Roy SW. Origin of spliceosomal introns and alternative splicing. Cold Spring Harb Perspect Biol 2014;6:a016071. [Crossref] [PubMed]
- Galej WP, Nguyen TH, Newman AJ, et al. Structural studies of the spliceosome: zooming into the heart of the machine. Curr Opin Struct Biol 2014;25:57-66. [Crossref] [PubMed]
- Hoskins AA, Moore MJ. The spliceosome: a flexible, reversible macromolecular machine. Trends Biochem Sci 2012;37:179-88. [Crossref] [PubMed]
- Liu S, Cheng C. Alternative RNA splicing and cancer. Wiley Interdiscip Rev RNA 2013;4:547-66. [Crossref] [PubMed]
- van der Feltz C, Anthony K, Brilot A, et al. Architecture of the spliceosome. Biochemistry 2012;51:3321-33. [Crossref] [PubMed]
- Will CL, Luhrmann R. Spliceosomal UsnRNP biogenesis, structure and function. Curr Opin Cell Biol 2001;13:290-301. [Crossref] [PubMed]
- Thanaraj TA, Clark F. Human GC-AG alternative intron isoforms with weak donor sites show enhanced consensus at acceptor exon positions. Nucleic Acids Res 2001;29:2581-93. [Crossref] [PubMed]
- Matera AG, Wang Z. A day in the life of the spliceosome. Nat Rev Mol Cell Biol 2014;15:108-21. [Crossref] [PubMed]
- Wahl MC, Will CL, Luhrmann R. The spliceosome: design principles of a dynamic RNP machine. Cell 2009;136:701-18. [Crossref] [PubMed]
- Fu XD, Ares M Jr. Context-dependent control of alternative splicing by RNA-binding proteins. Nat Rev Genet 2014;15:689-701. [Crossref] [PubMed]
- Will CL, Lührmann R. Spliceosome structure and function. Cold Spring Harb Perspect Biol 2011;3:a003707. [Crossref] [PubMed]
- Stine ZE, Walton ZE, Altman BJ, et al. MYC, Metabolism, and Cancer. Cancer Discov 2015;5:1024-39. [Crossref] [PubMed]
- Hirvonen H, Hukkanen V, Salmi TT, et al. Expression of L-myc and N-myc proto-oncogenes in human leukemias and leukemia cell lines. Blood 1991;78:3012-20. [PubMed]
- Zhu WM, Dong WF, Minden M. Alternate splicing creates two forms of the human kit protein. Leuk Lymphoma 1994;12:441-7. [Crossref] [PubMed]
- Piao X, Curtis JE, Minkin S, et al. Expression of the Kit and KitA receptor isoforms in human acute myelogenous leukemia. Blood 1994;83:476-81. [PubMed]
- Säily M, Koistinen P, Pulkki K, et al. Acute myeloblastic leukaemia cells produce soluble interleukin 6 receptor by a mechanism of alternative splicing. Cytokine 1998;10:860-7. [Crossref] [PubMed]
- Chong ZZ, Maiese K. The Src homology 2 domain tyrosine phosphatases SHP-1 and SHP-2: diversified control of cell growth, inflammation, and injury. Histol Histopathol 2007;22:1251-67. [PubMed]
- Beghini A, Ripamonti CB, Peterlongo P, et al. RNA hyperediting and alternative splicing of hematopoietic cell phosphatase (PTPN6) gene in acute myeloid leukemia. Hum Mol Genet 2000;9:2297-304. [Crossref] [PubMed]
- Chen X, Duan N, Zhang C, et al. Survivin and Tumorigenesis: Molecular Mechanisms and Therapeutic Strategies. J Cancer 2016;7:314-23. [Crossref] [PubMed]
- Carter BZ, Qiu Y, Huang X, et al. Survivin is highly expressed in CD34(+)38(-) leukemic stem/progenitor cells and predicts poor clinical outcomes in AML. Blood 2012;120:173-80. [Crossref] [PubMed]
- Zhou J, Bi C, Janakakumara JV, et al. Enhanced activation of STAT pathways and overexpression of survivin confer resistance to FLT3 inhibitors and could be therapeutic targets in AML. Blood 2009;113:4052-62. [Crossref] [PubMed]
- Adida C, Recher C, Raffoux E, et al. Expression and prognostic significance of survivin in de novo acute myeloid leukaemia. Br J Haematol 2000;111:196-203. [Crossref] [PubMed]
- Tamm I, Richter S, Oltersdorf D, et al. High expression levels of x-linked inhibitor of apoptosis protein and survivin correlate with poor overall survival in childhood de novo acute myeloid leukemia. Clin Cancer Res 2004;10:3737-44. [Crossref] [PubMed]
- Fukuda S, Abe M, Onishi C, et al. Survivin selectively modulates genes deregulated in human leukemia stem cells. J Oncol 2011;2011:946936. [Crossref] [PubMed]
- Mahotka C, Wenzel M, Springer E, et al. Survivin-deltaEx3 and survivin-2B: two novel splice variants of the apoptosis inhibitor survivin with different antiapoptotic properties. Cancer Res 1999;59:6097-102. [PubMed]
- Li F. Role of survivin and its splice variants in tumorigenesis. Br J Cancer 2005;92:212-6. [PubMed]
- Wagner M, Schmelz K, Wuchter C, et al. In vivo expression of survivin and its splice variant survivin-2B: impact on clinical outcome in acute myeloid leukemia. Int J Cancer 2006;119:1291-7. [Crossref] [PubMed]
- Badran A, Yoshida A, Ishikawa K, et al. Identification of a novel splice variant of the human anti-apoptopsis gene survivin. Biochem Biophys Res Commun 2004;314:902-7. [Crossref] [PubMed]
- Collins CT, Hess JL. Role of HOXA9 in leukemia: dysregulation, cofactors and essential targets. Oncogene 2016;35:1090-8. [Crossref] [PubMed]
- Alharbi RA, Pettengell R, Pandha HS, et al. The role of HOX genes in normal hematopoiesis and acute leukemia. Leukemia 2013;27:1000-8. [Crossref] [PubMed]
- Stadler CR, Vegi N, Mulaw MA, et al. The leukemogenicity of Hoxa9 depends on alternative splicing. Leukemia 2014;28:1838-43. [Crossref] [PubMed]
- Lopotová T, Polák J, Schwarz J, et al. Expression of four major WT1 splicing variants in acute and chronic myeloid leukemia patients analyzed by newly developed four real-time RT PCRs. Blood Cells Mol Dis 2012;49:41-7. [Crossref] [PubMed]
- Kramarzova K, Stuchly J, Willasch A, et al. Real-time PCR quantification of major Wilms' tumor gene 1 (WT1) isoforms in acute myeloid leukemia, their characteristic expression patterns and possible functional consequences. Leukemia 2012;26:2086-95. [Crossref] [PubMed]
- Siehl JM, Reinwald M, Heufelder K, et al. Expression of Wilms' tumor gene 1 at different stages of acute myeloid leukemia and analysis of its major splice variants. Ann Hematol 2004;83:745-50. [Crossref] [PubMed]
- Gu W, Hu S, Chen Z, et al. High expression of WT1 gene in acute myeloid leukemias with more predominant WT1+17AA isoforms at relapse. Leuk Res 2010;34:46-9. [Crossref] [PubMed]
- Grunwald MR, Levis MJ. FLT3 Tyrosine Kinase Inhibition as a Paradigm for Targeted Drug Development in Acute Myeloid Leukemia. Semin Hematol 2015;52:193-9. [Crossref] [PubMed]
- Zhou J, Khng J, Jasinghe VJ, et al. In vivo activity of ABT-869, a multi-target kinase inhibitor, against acute myeloid leukemia with wild-type FLT3 receptor. Leuk Res 2008;32:1091-100. [Crossref] [PubMed]
- Garg M, Nagata Y, Kanojia D, et al. Profiling of somatic mutations in acute myeloid leukemia with FLT3-ITD at diagnosis and relapse. Blood 2015;126:2491-501. [Crossref] [PubMed]
- Zhou J, Pan M, Xie Z, et al. Synergistic antileukemic effects between ABT-869 and chemotherapy involve downregulation of cell cycle-regulated genes and c-Mos-mediated MAPK pathway. Leukemia 2008;22:138-46. [Crossref] [PubMed]
- Zhou J, Goh BC, Albert DH, et al. ABT-869, a promising multi-targeted tyrosine kinase inhibitor: from bench to bedside. J Hematol Oncol 2009;2:33. [Crossref] [PubMed]
- Zhou J, Bi C, Chng WJ, et al. PRL-3, a metastasis associated tyrosine phosphatase, is involved in FLT3-ITD signaling and implicated in anti-AML therapy. PLoS One 2011;6:e19798. [Crossref] [PubMed]
- Kiyoi H, Yanada M, Ozekia K. Clinical significance of FLT3 in leukemia. Int J Hematol 2005;82:85-92. [Crossref] [PubMed]
- Adamia S, Haibe-Kains B, Pilarski PM, et al. A genome-wide aberrant RNA splicing in patients with acute myeloid leukemia identifies novel potential disease markers and therapeutic targets. Clin Cancer Res 2014;20:1135-45. [Crossref] [PubMed]
- Adamia S, Bar-Natan M, Haibe-Kains B, et al. NOTCH2 and FLT3 gene mis-splicings are common events in patients with acute myeloid leukemia (AML): new potential targets in AML. Blood 2014;123:2816-25. [Crossref] [PubMed]
- Shaffer BC, Gillet JP, Patel C, et al. Drug resistance: still a daunting challenge to the successful treatment of AML. Drug Resist Updat 2012;15:62-9. [Crossref] [PubMed]
- Thol F, Schlenk RF, Heuser M, et al. How I treat refractory and early relapsed acute myeloid leukemia. Blood 2015;126:319-27. [Crossref] [PubMed]
- Tallman MS, Gilliland DG, Rowe JM. Drug therapy for acute myeloid leukemia. Blood 2005;106:1154-63. [Crossref] [PubMed]
- Veuger MJ, Honders MW, Landegent JE, et al. High incidence of alternatively spliced forms of deoxycytidine kinase in patients with resistant acute myeloid leukemia. Blood 2000;96:1517-24. [PubMed]
- Veuger MJ, Heemskerk MH, Honders MW, et al. Functional role of alternatively spliced deoxycytidine kinase in sensitivity to cytarabine of acute myeloid leukemic cells. Blood 2002;99:1373-80. [Crossref] [PubMed]
- Mohamed AM, Balsat M, Thenoz M, et al. Oncogene- and drug resistance-associated alternative exon usage in acute myeloid leukemia (AML). Oncotarget 2016;7:2889-909. [PubMed]
- de Necochea-Campion R, Shouse GP, Zhou Q, et al. Aberrant splicing and drug resistance in AML. J Hematol Oncol 2016;9:85. [Crossref] [PubMed]
- Malcovati L, Papaemmanuil E, Bowen DT, et al. Clinical significance of SF3B1 mutations in myelodysplastic syndromes and myelodysplastic/myeloproliferative neoplasms. Blood 2011;118:6239-46. [Crossref] [PubMed]
- Papaemmanuil E, Gerstung M, Bullinger L, et al. Genomic Classification and Prognosis in Acute Myeloid Leukemia. N Engl J Med 2016;374:2209-21. [Crossref] [PubMed]
- Jiang Y, Zhu Y, Liu ZJ, et al. The emerging roles of the DDX41 protein in immunity and diseases. Protein Cell 2017;8:83-9. [Crossref] [PubMed]
- Polprasert C, Schulze I, Sekeres MA, et al. Inherited and Somatic Defects in DDX41 in Myeloid Neoplasms. Cancer Cell 2015;27:658-70. [Crossref] [PubMed]
- Lewinsohn M, Brown AL, Weinel LM, et al. Novel germ line DDX41 mutations define families with a lower age of MDS/AML onset and lymphoid malignancies. Blood 2016;127:1017-23. [Crossref] [PubMed]
- Berger G, van den Berg E, Sikkema-Raddatz B, et al. Re-emergence of acute myeloid leukemia in donor cells following allogeneic transplantation in a family with a germline DDX41 mutation. Leukemia 2017;31:520-2. [Crossref] [PubMed]
- Grainger RJ, Beggs JD. Prp8 protein: at the heart of the spliceosome. RNA 2005;11:533-57. [Crossref] [PubMed]
- Keightley MC, Crowhurst MO, Layton JE, et al. In vivo mutation of pre-mRNA processing factor 8 (Prpf8) affects transcript splicing, cell survival and myeloid differentiation. FEBS Lett 2013;587:2150-7. [Crossref] [PubMed]
- Kurtovic-Kozaric A, Przychodzen B, Singh J, et al. PRPF8 defects cause missplicing in myeloid malignancies. Leukemia 2015;29:126-36. [Crossref] [PubMed]
- Shav-Tal Y, Zipori D. PSF and p54(nrb)/NonO--multi-functional nuclear proteins. FEBS Lett 2002;531:109-14. [Crossref] [PubMed]
- Knott GJ, Bond CS, Fox AH. The DBHS proteins SFPQ, NONO and PSPC1: a multipurpose molecular scaffold. Nucleic Acids Res 2016;44:3989-4004. [Crossref] [PubMed]
- Dolnik A, Engelmann JC, Scharfenberger-Schmeer M, et al. Commonly altered genomic regions in acute myeloid leukemia are enriched for somatic mutations involved in chromatin remodeling and splicing. Blood 2012;120:e83-92. [Crossref] [PubMed]
- Liu J, Huang B, Xiao Y, et al. Aberrant expression of splicing factors in newly diagnosed acute myeloid leukemia. Onkologie 2012;35:335-40. [Crossref] [PubMed]
- Zeichner SB, Arellano ML. Secondary Adult Acute Myeloid Leukemia: a Review of Our Evolving Understanding of a Complex Disease Process. Curr Treat Options Oncol 2015;16:37. [Crossref] [PubMed]
- Larson RA. Is secondary leukemia an independent poor prognostic factor in acute myeloid leukemia? Best Pract Res Clin Haematol 2007;20:29-37. [Crossref] [PubMed]
- Crews LA, Balaian L, Delos Santos NP, et al. RNA Splicing Modulation Selectively Impairs Leukemia Stem Cell Maintenance in Secondary Human AML. Cell Stem Cell 2016;19:599-612. [Crossref] [PubMed]
- Bonnal S, Vigevani L, Valcarcel J. The spliceosome as a target of novel antitumour drugs. Nat Rev Drug Discov 2012;11:847-59. [Crossref] [PubMed]
- Lee SC, Abdel-Wahab O. Therapeutic targeting of splicing in cancer. Nat Med 2016;22:976-86. [Crossref] [PubMed]
- Villa R, Mandel AL, Jones BD, et al. Structure of FD-895 revealed through total synthesis. Org Lett 2012;14:5396-9. [Crossref] [PubMed]
Cite this article as: Zhou J, Chng WJ. Aberrant RNA splicing and mutations in spliceosome complex in acute myeloid leukemia. Stem Cell Investig 2017;4:6.