Endothelial progenitor cells in hematologic malignancies
Endothelial progenitor cells (EPCs)
Angiogenesis and vasculogenesis are two different biologic processes, through which new blood vessels are formed to support oxygen and nutrient supply to distant tissues. The term angiogenesis denotes a biological process involving the formation of new blood vessels from pre-existing ones, while vasculogenesis is the term used to indicate the formation of new blood vessels from no pre-existing ones. During embryogenesis, new blood vessel formation is strictly required to sustain organ development and during tissue reparation new blood vessel formation is required to sustain the survival of the repairing tissue. In some pathological conditions the angiogenetic processes are involved in physiopathological processes related to many human pathologic conditions, such as ischemic disorders or neoplastic diseases.
The discovery of EPCs in adult tissues has challenged the view that new vessel formation occurs only during embryonic life. Thus, new blood vessels in the adults have been considered to be generated not only through an angiogenetic process, but also via postnatal vasculogenesis driven by EPCs. However, it is still a matter of great debate and controversy as to what extent EPCs may really contribute to new vessel formation during adult life.
The current evidences indicate that cells exhibiting the functional property of EPCs are observed at the level of the vessel wall, in the blood and in some hemopoietic tissues.
Evidences published in the last years strongly suggest the existence of different types of stem/progenitor cells localized at the level of the vessel wall, collectively identified as vascular wall-resident stem cells (VW-SCs) (1). Particularly, the current studies suggest the existence of four different types of stem/progenitor cells, with different proliferative and differentiative capacities, resident at the level of the adventitia of the vascular wall layer: EPCs, mesenchymal stem cells, smooth muscle progenitor cells and pericytes (1). EPCs have been identified at the level of vessels of large and intermediate size, localized in the luminal endothelium and in the inner lining of adventitia (2). These authors also showed that human umbilical vein endothelial cells (HUVEC) and human aortic endothelial cells (HAECs), although considered to be differentiated endothelial cells, are capable of at least 40 population doublings in vitro and are in fact composed by a mixture of EPCs with low and high proliferative potential (2). Other studies have identified in human arteries a distinct zone of the vascular wall, localized between smooth muscle and adventitial layer, containing predominantly CD34+, CD31−, Tie2+ and VEGFR2+ cells, largely CD45−: these cells are able to migrate and to form new vessels (3). A vascular progenitor cell was identified also in the walls of coronary arteries: these cells were identified as c-kit+/VEGF-R2+ cells and are capable of self-renewing and differentiation into endothelial cells, smooth muscle cells and partly into cardiomyocytes (4). In a dog stenotic artery model, these cells were shown to be capable in vivo of coronary artery regeneration (4). These findings were confirmed through studies carried out in normal mice, providing evidence about the existence of a side population of CD31+CD45− endothelial cells present in the inner surface of blood vessels and able to induce in vivo the reconstitution of durable, functioning blood vessels in ischemic milieu (5,6).
A large number of studies carried out in these last years was focused to identify and to characterize cells endowed with a potential endothelial progenitor cell activity present at the level of hematopoietic tissues or circulating in the blood. In this research area an initial seminal paper by Asahara and coworkers introduced the first scientific demonstration on the presence of an endothelial progenitor cell present in circulation and capable of de novo blood vessel formation (7). Since this initial observation, there were many studies in this field that have originated a great debate concerning the definition and characterization of what can be considered as real EPCs. The complexity of the field was also driven by the consistent heterogeneity of the methodology used to characterize these cells and to try to obtain their purification (8,9).
Basically, these studies led to the identification of two types of EPCs: (I) proangiogenic hematopoietic cells, corresponding to cells of hematopoietic origin that promote angiogenesis via paracrine effects; (II) endothelial colony forming cells (ECFCs) that are able to generate a progeny of phenotypically and functionally competent endothelial cells able to form vessels in vivo (Table 1). Basically, two types of proangiogenic hematopoietic cells have been identified, one with a more mature phenotype and corresponding to mature Tie2+ monocytic cells and an immature progenitor cell population, corresponding to proangiogenic hematopoietic progenitor cells or CFU-Hill (17). The studies carried out in these last years on proangiogenic hematopoietic cells have led to the conclusion that these cells derive from the differentiation of a subset of hematopoietic progenitor cells, characterized by positivity for CD34, CD133 and VEGF-R2, mobilized from bone marrow by angiogenic growth factors and contributing in vivo to an angiogenetic response only through an indirect effect based on paracrine mechanisms (18-21).
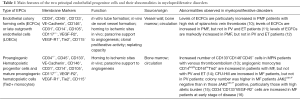
Full table
Another assay allows the growth of true endothelial cells and is called the outgrowth endothelial cells (OECs): this assay identifies endothelial progenitors exhibiting clonal endothelial colony-forming cell (ECFS) capacity, giving rise in vitro to the formation of large colonies of human endothelial CD45− cells within 1–3 weeks of culture, when blood cells are plated on culture dishes coated with matrix proteins (22). In 2004, Ingram and coworkers have improved this methodology by growing Ficoll-isolated mononuclear cells resuspended in endothelial cell culture medium EGM-2; after 24 h of culture, the non-adherent cells were removed and the adherent cells were grown in the same medium (23). After 1–3 weeks of culture, areas of growth of endothelial cells are observed under form of circumscribed monolayers of cobblestone-appearing cells (24). These outgrowth endothelial colonies are very rare in that their number in the normal PB was estimated to be less than 1 colony/20 mL of blood, while their number is higher in cord blood (about 8 colonies/20 mL of blood). The antigenic profile of the cell progeny observed in these colonies corresponds to a typical endothelial phenotype (24). Subsequent studies provided evidence that there are different types of EPC-derived endothelial cells that could be discriminated according to their variable cell autonomous proliferative potential and that EPC-derived endothelial cells display a hierarchy of proliferative capacity, reminiscent of the hematopoietic progenitor cell hierarchy (25). As it is expected, cord blood EPC-derived endothelial cells display a higher proliferative potential than those derived from adult PB endothelial progenitors (25). According to these findings, Yoder and coworkers have proposed a new classification of endothelial progenitor cells (26).
Subsequent studies have provided clear evidence that CD34+/CD45− cells, but not CD34+/CD45+ cells are able to generate ECFCs (27). Interestingly, CD34+/CD45+ cells are only able to generate under the endothelial cell culture conditions monocytic cells (27). In this study it was also shown that CD133+ cells are unable to generate ECFCs (27).
A recent study by Tura and coworkers provided additional data to better understand the origin of circulating ECFCs (28). In fact, these authors provided evidence that ECFCs are observed both in PB and CB (in agreement with many previous reports), but are not detectable in bone marrow and in mobilized PB (28). ECFCs were considerably enriched in CD34+/CD133− and, particularly in CD34+/CD146+/CD133− cells, compared to their frequency observed in total CD34+ cells (28). Importantly, CD34+/CD146+/CD133− cells isolated from bone marrow failed to generate ECFCs (28). According to these findings it was suggested that ECFCs are not generated from bone marrow and, seemingly, are not of hematopoietic origin (28).
Since the discovery of ECFCs there was significant interest in their therapeutic impact to treat vascular injuries. Several recent reports indicate that high proliferative late outgrowth endothelial progenitors are capable in vivo of repairing vascular damage in models of ischemic hind limbs (29), pulmonary artery hypertension (30) and myocardial infarction (31).
Do endothelial cells belong to the primitive leukemic cell clone in myeloproliferative disorders?
Myeloproliferative neoplasms (MPNs) are a heterogeneous group of clonal hematopoietic stem cell disorders, characterized by significant increases in one or more myeloid cell lineages. Chronic myeloid leukemia (CML) is a well-characterized MPN originated from a reciprocal translocation between chromosomes 9 and 22, determining the formation and the expression of the BCR-ABL fusion gene. The pathophysiology of this MPN is at a large extent dictated by the expression and the biologic activity of the chimeric BCR-ABL protein. In contrast, Philadelphia chromosome-negative MPNs include polycythemia vera (PV), essential thrombocythemia (ET) and primary myelofibrosis (PMF) and are characterized by the frequent occurrence of mutations in the Janus kinase 2 (JAK2) and MPL genes.
Chronic myeloid leukemia (CML)
Several studies carried out in CML suggest that in this disease endothelial cells could belong to the leukemic clone. Thus, Gunsilius and coworkers have provided evidence about the presence of the fusion BCR-ABL transcript in endothelial cells derived in vitro from bone marrow progenitor cells (32). These findings were interpreted as possible evidence that CML originates from a bone marrow-derived hemangioblast progenitor cell able to generate both blood cells and endothelial cells (1). Subsequent studies have provided conflicting results in that Wu et al. isolated CD31+/CD105+ cells from bone marrow of six CML patients and showed that the majority of these cells display the BCR-ABL transcript (33), while Otten et al. have grown in vitro outgrowth endothelial cells from 19 CML patients and have failed to detect BCR-ABL transcripts in these endothelial cells generated in vitro from circulating endothelial progenitors (34).
Other evidences were based on the isolation of VEGF-R2+ cells from the bone marrow of CML patients: these cells express the fusion transcript and are able to generate in vitro endothelial cells (35). Additional evidences showed that VEGF-R2+CD34− cells in bone marrow of CML patients could act as multipotent progenitor cells, generating also endothelial cells (36).
These evidences represent still only a preliminary suggestion and more definitive proofs seem to be required to definitely demonstrate that endothelial cells belong to the leukemic clone in CML.
Although it is still unclear the involvement of endothelial lineage in the CML pathogenetic process, it was clearly demonstrated that in CML, as well in other MPNs, there is an increased bone marrow vascularity, as evidenced at histological level by increased vessel density, with tortuous vessel architecture and increased branching; furthermore, in CML bone marrow an increased number of VEGF+ cells was observed (37). A more recent study confirmed this finding and showed also that the level of bone marrow microvascular density (MVD) is increased in CML, but it is not affected by the different phases of development of the disease, histological subtypes of CML and grades of medullary fibrosis (38).
Various biochemical mechanisms could be responsible for the activation of a neoangiogenetic response in CML and these mechanisms are seemingly related to the release by leukemic cells of molecules with pro-angiogenetic activities. Recent studies indicate that CML cells release extracellular vesicles (EVs) able to stimulate the process of vascularization by human vascular endothelial cells (39), stimulating the expression of both ICAM-1 and VCAM-1 adhesion molecule (40). The proangiogenic activity of exosomes released by CML cells can be pharmacologically modulated: thus, the anticancer drug curcumin considerably attenuates the exosome’s ability to promote an angiogenic phenotype, by preferentially shutting miR-21 in these microvesicles (41).
Interestingly, a recent study provided preliminary evidence that EVs released by CML cells can mediate the transfer of BCR-ABL mRNA to endothelial cells (42).
This finding challenges the hypothesis that endothelial cells may be part of the Philadelphia+ clone in CML (42). BCR-ABL could transfer from CML cells to endothelial cells also through another mechanism involving the transfer of DNA fragments (43). Exosomes purified from K562 cells are able to transfer in vitro and in vivo DNA fragments containing BCR-ABL (44). However, these are only very preliminary evidences and additional carefully controlled experiments are required to assess whether or not the transferred BCR/ABL DNA has pathophysiological significance.
Philadelphia-negative MPNs
Several studies were performed in the last years to define a possible direct implication of the endothelial lineage in the pathologic process involving JAK2V617F-mutated MPNs. These studies are particularly important because Philadelphia-negative MPNs show a high incidence of vascular complications. Initial studies based on in vitro assays for endothelial progenitors showed that JAK2V617F mutations were identified only in CFU-ECs (colonies formed by monocytoid cells with pro-angiogenic activity), but not in E-CFCs (colonies by true endothelial cells) (11). At variance with these findings, Sozer and coworkers reported that endothelial cells isolated by microdissection from liver biopsies of patients with PV with Budd-Chiari syndrome exhibit the JAK2V617F mutation (45).
Given these conflicting evidences, Teofili and coworkers have re-explored this issue, reporting the analysis of the MPN-related markers in E-CFCs generated from 22 Ph− MPN patients and they observed that in about 55% of cases endothelial cells do not display molecular abnormalities, while in remaining 45% of patients MPN-related abnormalities have been observed, including either SOCS gene hypermethylation or JAK2V617F mutations. Interestingly, endothelial cells with JAK2V617F mutation displayed a Stat3/Stat5 activation higher than that observed in endothelial cells from healthy subjects. Importantly, all the patients with molecular abnormalities at the level of the endothelial lineage experienced thrombotic complications (12). The possible involvement of the endothelial cell lineage in the process of malignant transformation in Ph− MPN was further supported by another recent study investigating spleen endothelial cells in myelofibrosis patients (46). Thus, Rosti and coworkers showed that endothelial cells carrying the JAK2V617F mutation can be detected in >60% of myelofibrosis patients in the splenic capillaries and in the splenic vein (46). It was hypothesized that the mutated endothelium may be involved in the malignant transformation of myelofibrosis.
The simplest interpretation of these observations is based on the hypothesis that a common progenitor cell of the hematopoietic and endothelial lineages, the hemangioblast, could represent the cellular target of the leukemogenetic transformation. Alternative hypotheses have been proposed related to the acquisition of external DNA into endothelial cells in patients with MPNs, following events of cell fusion or of DNA acquisition from the blood.
As observed in CML, also in the bone marrow of Philadelphia-negative MPN there is evidence of an increased vascularity, this increase being particularly pronounced in myelofibrosis (47). The increased MVD observed in these MPNs was found to be correlated with VEGF levels and was higher in PV and MF than in ET (47). Other investigators observed a strong correlation between VEGF levels, MVD and JAK2V617F mutant allele burden (48). Finally, Boiocchi and coworkers reported increased expression of VEGF-R1, correlating with VEGF and MVD in the bone marrow of Ph− MPNs, with a decreasing gradient MF > PV > ET (49). VEGF and VEGF-R1 were increased and co-localized in megakaryocytes, macrophages and myeloid precursors (49).
In addition to the possible involvement of the endothelial cell lineage in the process of clonal development of Ph− MPNs, several studies have explored the number of ECFCs in these disorders (Table 1). Basically, these studies have shown that ECFCs are increased in MF, but not in PV and ET (11,12). Furthermore, the levels of ECFCs are particularly increased in MF patients with high risk of splanchnic vein thrombosis (10). Other studies have analyzed modifications of the number of various types of proangiogenic hematopoietic progenitors in Ph− MPN patients. Essentially these studies have shown increased numbers of CD34+CD133+VEGF-R2+ cells (16) or CD133+CD146+CD45+ cells (13) predominantly occurring in patients with venous thromboembolism. Angiogenic monocytes CD14brightCD16lowTie2+ are increased in patients with MF, but not with PV or ET (14). Finally, Sozer et al. have reported an increased number of CFU-Hill coloniesw in MF, but not in PV patients: colony number was higher in patients JAK2V617 negative than in those JAK2V617F positive, particularly those with high allelic burden (15).
Angiogenesis in acute myeloid leukemias (AML)
Angiogenesis, the process of blood vessel formation, is necessary for the survival of tissutal cells both in normal and pathological conditions. Increased angiogenesis is an event currently observed in many hematologic malignancies. Particularly, in AMLs, increased angiogenesis in bone marrow niche is correlated with leukemia progression and resistance to treatment (50). The large majority of the AML bone marrow biopsies exhibited higher VEGF-A protein expression levels than normal bone marrow (51,52). The highest VEGF-A mRNA expression levels are observed among t(8;21) and t(15;17) translocated AML patients (53,54). These findings were recently confirmed by Saulle and coworkers through an analysis of TCGA data, clearly showing that the highest VEGF-A levels are observed at the level of M3 t(15;17) translocated AMLs (55). Recent studies have in part clarified the molecular mechanisms responsible for the elevated VEGF-A levels in these AML subtypes. Thus Ter Elst and coworkers have shown that the formation of the AML1-ETO disrupts the suppressive effect of the AML1 transcription factor on VEGF-A expression (54). On the other hand, Saulle and coworkers have shown that PML-RARα, the fusion protein formed as a consequence of the t(15;17) translocation, induces a significant downmodulation of the expression of HHEX, a homeobox transcription factor exerting a repressive effect on VEGF-A gene expression (55). The highest VEGF-R1 levels were observed in t(15;17) translocated AMLs (53). The highest VEGF-R2 levels were observed in t(8;21) translocated AMLs (31) and in MLL-rearranged AML samples (56).
Other studies have shown that CD7+ AMLs (an AML subset characterized by the inappropriate expression of the T-cell antigen CD7 and often corresponding to FLT3-mutated AMLs) are characterized by the high expression of Angiopoietin-2 (Ang-2) (57). Riccioni and coworkers have identified a subset of AMLs characterized by the elevated expression of the Ang-2 receptor Tie-2 and by the expression of these VEGF-Rs: these AMLs often have a monocytic phenotype and are frequently FLT3-mutated (58). In these AMLs a Ang1/Tie-2 autocrine loop was identified, required for leukemic blast survival/proliferation (58). There is evidence that for some AML subsets, blast cell proliferation is partially dependent on the VEGF/VEGFR signaling. This is particularly true for t(8;21) and MLL-rearranged AMLs, in which VEGF-R2 kinase inhibition elicited an inhibitory effect on leukemic cell survival, while this effect was not observed in t(15;17) AML samples (59). Experiments of enforced VEGF-R2 expression in the TF1 leukemic cell line provided evidence that this receptor was able to sustain the survival and proliferation of leukemic cells (60).
Histological studies provided evidence about increased angiogenesis in the bone marrow of AML patients. Immunohistological studies have provided evidence about an increased MVD in the bone marrow of AML patients; induction of disease remission with chemotherapy was associated with a clear decrease of MVD; in patients not achieving complete remission, MVD remained high (61,62). Immunohistochemical analyses of bone marrow biopsies from APL patients provided evidence about a clear increase of MVD and VEGF levels; treatment with all-trans retinoic acid (ATRA) inhibited VEGF production and suppressed angiogenesis (63).
Recent studies have explored the effects of leukemic cells on endothelial cell biology through a co-culture strategy. These studies have shown that AML cells regulate angiogenesis by secreting soluble factors (mainly represented by VEGF) that enhance endothelial cell function, as well as by direct cellular interactions with endothelial cells (64). The cellular interaction between AML cells and endothelial cell promotes Notch/DLL4 pathway activation (64). The NOTCH/DLL4 pathway activation could result in an inhibitory effect on angiogenesis, which is prevented by VEGF (64).
Interestingly, Shih and coworkers have developed a non-invasive dynamic contrast-enhanced magnetic resonance imaging technique to monitor the MVD at the level of the lumbar vertebrae and correlated this parameter to treatment outcome in AML: patients with higher contrast values, underlying higher MVD values, had less chance to achieve complete responses, compared to patients with lower contrast values (65).
The co-culture experiments using primary AML cells and microvascular endothelial cells have shown that the leukemic blasts are able to secrete numerous cytokines that increase the proliferation (66) and the tubulogenic function of endothelial cells (67); on the other hand, endothelial cells secrete factors that improve the survival and proliferation (68) and reduce the chemosensitivity (69) of AML cells. Interestingly, leukemia-derived endothelial cells are more prone than normal endothelial cells to sustain the survival and proliferation of leukemic cells (70).
Cogle and coworkers have made a very intriguing observation concerning the functional integration of AML cells in blood vessels (71). In fact, through the analysis of autoptic tissues and studying animal models of human AMLs, it was reached the conclusion that AML cells are able to integrate within the vascular endothelium (71). Intriguingly, AML cells after endothelial differentiation in vitro are able, when injected in vivo, to develop clusters of AML cells in the bone marrow of recipient animals (71).
Given all these observations, various experimental and clinical studies are in progress to try to potentiate the response of AML cells to standard chemotherapy either using agents that mobilize leukemic cells away from the protective vascular niche or agents that disrupt the bone marrow vascular niches (72).
Angiogenesis in myelodysplastic syndromes
Myelodysplastic syndromes (MDSs) are a group of heterogeneous clonal hematopoietic disorders characterized by ineffective hematopoiesis and a tendency to transform to acute leukemias. As in other hematologic malignancies also in MDSs an increased bone marrow angiogenesis is observed. Thus, immunohistochemical studies have evidenced an increased MVD in the bone marrow specimens of MDS patients compared to normal bone marrow (73-76). The results concerning the correlation between bone marrow MVD and the stage of disease have provided conflicting evidences: in fact, some studies have reported a higher MVD preferentially among high-risk MDS patients (73,76), while other studies have failed to find such a correlation (77). Similarly, the prognostic significance of MVD in MDS patients remains unclear (73,76-78). Surprisingly, during the transformation of MDSs to overt leukemic disease, a decrease of MVD was observed, associated with a switch from the predominant production in the bone marrow of pro-angiogenic cytokines, such as VEGF, bFGF, TNF-α, HGF, Ang-1 and Ang-2 to the production of the anti-angiogenic cytokine TGF-β (79). Some of the pro-angiogenic cytokines released in the bone marrow microenvironment seem to have a prognostic impact. Thus, Cheng and coworkers have investigated the potential prognostic impact of various pro-angiogenic cytokines and have shown that a higher Ang-1 expression in the bone marrow is an independent poor prognostic factor for overall survival in MDS patients, irrespective of age, karyotype and disease score (80). Furthermore, patients with higher Ang-1 levels have a higher chance of AML transformation (80).
Some recent studies have explored both circulating endothelial cells (CECs) and endothelial progenitor cells in MDSs. The results of these studies provided evidence that the number of both CECs and ECFCs is consistently increased in MDSs (81,82). Particularly, Dalla Porta and coworkers observed an increased number of CECs, their number being positively correlated with bone marrow MVD and negatively correlated with the international prognostic scoring system risk (81). Intriguingly, these authors showed that a variable, but significant proportion of CECs display the same chromosome abnormalities detected at the level of the hematopoietic cells (81). Teofili and coworkers observed that the levels of ECFCs is markedly increased in low-risk MDS patients, compared to normal controls (82); furthermore, ECFCs derived from MDSs display several epigenetic abnormalities and were less competent than those derived from normal controls to support normal hematopoiesis from CD34+ cells (82).
Angiogenesis in multiple myeloma
Angiogenesis is an event constantly associated with multiple myeloma progression and has important prognostic implications. The increased angiogenesis observed in this disease is related to the peculiar tumor microenvironment dictated by the interaction of tumor plasma cells with surrounding host cells and extracellular matrix. One of the main mechanisms responsible for the increased angiogenesis is related to the release of angiogenic cytokines directly by tumoral plasma cells or by cells present in the tumor microenvironment (83). In this context, initial studies have provided clear evidence that an increased angiogenesis characterizes multiple myeloma development from the stage of monoclonal gammopathy of undetermined significance, to non-active multiple myeloma and, finally, to active multiple myeloma (84). This conclusion was reached through immunohistological studies and through the characterization of the angiogenic activity of cytokines released by tumoral cells isolated from specimens corresponding to disease at various stages of development (84). Impressive were the results of a study based on the analysis of 400 bone marrow specimens derived from patients with disease at various stages of development. The results of this study showed that MVD was 1.3 in normal bone marrow, 1.7 in primary amyloidosis, 3 in monoclonal gammopathy, 4 in smoldering multiple myeloma; 11 in primary diagnosed multiple myeloma and 20 in relapsing multiple myeloma (85). These results unequivocally show that angiogenesis increases with disease progression (85). Importantly, studies of evaluation of MVD and angiogenesis index in newly diagnosed multiple myeloma patients showed the clear and strong prognostic value of this parameter (86).
The tumor progression was accompanied not only by an increased production of pro-angiogenic cytokines by tumor plasma cells, but also by a decrease of molecules exerting an inhibitory activity on angiogenesis (87). The progressive growth of tumoral plasma cells inevitably exposes these cells to a condition of tissutal hypoxia, thus activating the hypoxia signaling pathway and the HIF-1α transcription factor, with consequent activation of numerous target genes. Among these HIF-1α target genes there are several angiogenic cytokines, including VEGF (88), angiopoietin-1 (89), osteopontin (90) and adrenomedullin (91).
Other studies have explored endothelial cells in multiple myeloma. Thus, Vacca and coworkers have isolated endothelial cells from the bone marrow of multiple myeloma patients and studied the main characteristics of these cells, including their heterogeneous phenotype, their pronounced angiogenic activity in vitro and in vivo, their high capacity to secrete growth and invasive factors active on plasma cells, their abnormal morphology and activated metabolic condition (92). Other studies have explored and characterized CECs (Defined as CD146+, CD105+, CD34+ and CD11c− cells) in multiple myeloma, showing that the number of these cells is increased in multiple myeloma compared to healthy controls (about a mean six fold increase) (93). In parallel, it was shown the presence of EPCs in the blood of multiple myeloma patients, whose number was decreased following thalidomide treatment (93). Rigolin and coworkers have characterized CECs in multiple myeloma patients with a clonal tumor marker (13q14 deletion): they observed that 11–32 CECs, isolated from 5 multiple myeloma patients with these features have the neoplastic clonal marker; furthermore, the large majority of CECs with the 13q14 deletion displayed properties of endothelial progenitors, as indicated by their positivity for CD133 antigen (94). In contrast, CECs isolated from patients with monoclonal gammopathy of undetermined significance with 13q14 deletion are cytogenetically normal (94). According to these observations it was hypothesized a possible origin of CECs from a common hemangioblast progenitor, able to generate both plasma cells and endothelial cells (94).
Recent studies suggest an important role of exosomes in the multiple myeloma progression. In the bone marrow microenvironment there is the release of exosomes by various types of cells. Thus, exosomes released by bone marrow mesenchymal stromal cells (BMMSCs) facilitate myeloma progression; this property is specifically displayed by BMMSCs present in the multiple myeloma bone marrow, but not in normal bone marrow (95). Importantly, exosomes released by multiple myeloma cells play an important role as stimulators of angiogenesis in the bone marrow microenvironment. Thus, it was shown that multiple myeloma chronically grown under hypoxic conditions release exosomes able to stimulate a robust angiogenetic effect: a key mediator of this effect is miR-135b, abundantly expressed in hypoxic multiple myeloma exosomes and targeting factor-inhibiting hypoxia-inducible factor 1 (FIH-1) in endothelial cells and resulting in HIF-1α activation and induction of an angiogenetic gene expression (96).
A recent study confirmed the angiogenic activity of myeloma-derived exosomes, suggesting that these microvesicles vehiculate a variety of angiogenic cytokines into endothelial cells (97). These studies showed that multiple myeloma exosomes display pro-angiogenic activity both in vitro and in vivo.
Given these observations, a recent study addressed the important problem of investigating the contribution of increased angiogenesis to tumor progression in myeloma multiple mouse models. Thus, Moschetta and coworkers have explored the role of vasculogenesis in a mouse model of multiple myeloma. First, an early mobilization of EPCs from bone marrow to peripheral blood, followed by recruitment to multiple myeloma-colonized niches, was observed; second, the use of ID1/ID3-negative mice, EPC-defective, showed that EPC trafficking is required for multiple myeloma progression; third, angiogenic dependency of multiple myeloma occurs at early, and not at late stages of tumor development; fourth, early targeting of EPCs with anti-VEGF-R2 antibody at early stages, but not at late stages of multiple myeloma development, delayed tumor progression (98). According to these observations it was observed that inhibition of angiogenesis at an early stage of disease may be studies in clinical trials of patients with smoldering multiple myeloma (98).
Conclusions and future perspectives
A better understanding of the mechanisms controlling angiogenesis during adult life is of fundamental importance because angiogenestic mechanisms play a key role in the tissue homeostasis and may play an essential role in tissue repair. Studies carried out in the last years underline a potentially important role of EPCs in the control of adult angiogenesis and vasculogenesis. However, in spite the progresses made in the identification and assay of the functional properties of EPCs, the basic cell biology of these cells is still scarcely defined and their physiologic role still remains the object of debate.
An increased angiogenesis is observed in many tumors, including hematologic malignancies and plays an important role in tumor progression. Various mechanisms are responsible for the increased angiogenesis observed in these tumors and involve also an increased recruitment of EPCs at the level of tumor development in the bone marrow. However, a better understanding of these mechanisms is required to define an efficient strategy to therapeutically inhibit the EPCs-mediated tumoral angiogenesis. The discovery of increased MVD in many hematologic malignancies has opened the way to anti-angiogenesis therapies (based on the use of three different agents: anti-angiogenic agents, vascular disrupting agents and leukemia mobilizing agents); however, these therapies, despite being effective, in the majority of patients failed to halt tumor progression. In future studies it would be of fundamental importance to determine an optimal target of these anti-EPC therapies and to define subpopulations of patients and appropriate stages of tumor development to be treated. The basic assumption of these studies is that inhibition of angiogenesis may improve the response of leukemic cells to standard chemotherapy and, then, patient survival.
Finally, several studies have suggested that in some hematological malignancies, such as MPNs, endothelial cells, could, at least in part, derive from the malignant clone and this observation has important implications for the understanding of the cellular origin of these neoplasia and for the understanding of the pathogenetic mechanisms underlying the increased and dysregulated angiogenesis observed in these conditions.
Acknowledgements
This work was supported by a grant of Italian Health Ministery, “Programma Oncotecnologico” to U Testa.
Footnote
Conflicts of Interest: The authors have no conflicts of interest to declare.
References
- Bobryshev YV, Orekhov AN, Chistiakov DA. Vascular stem/progenitor cells: current status of the problem. Cell Tissue Res 2015;362:1-7. [Crossref] [PubMed]
- Ingram DA, Mead LE, Woodard W, et al. Vessel wall-derived endothelial cells rapidly proliferate because they contain a complete hierarchy of endothelial progenitor cells. Blood 2005;105:2783-6. [Crossref] [PubMed]
- Zengin E, Chalajour F, Gehling UM, et al. Vascular wall resident progenitor cells: a source for postnatal vasculogenesis. Development 2006;133:1543-51. [Crossref] [PubMed]
- Bearzi C, Leri A, Lo Monaco F, et al. Identification of a coronary vascular progenitor cell in the human heart. Proc Natl Acad Sci U S A 2009;106:15885-90. [Crossref] [PubMed]
- Fang S, Wei J, Pentinmikko N, Leinonen H, et al. Generation of functional blood vessels from a single c-kit+ adult vascular endothelial stem cell. PLoS Biol 2012;10:e1001407. [Crossref] [PubMed]
- Naito H, Kidoya H, Sakimoto S, et al. Identification and characterization of a resident stem/progenitor cell population in pre-existing blood vessels. EMBO J 2012;31:842-55. [Crossref] [PubMed]
- Asahara T, Murohara T, Sullivan A, et al. Isolation of putative progenitor endothelial cells for angiogenesis. Science 1997;275:964-7. [Crossref] [PubMed]
- Pelosi E, Castelli G, Testa U. Endothelial progenitors. Blood Cells Mol Dis 2014;52:186-94. [Crossref] [PubMed]
- Basile DP, Yoder MC. Circulating and tissue resident endothelial progenitor cells. J Cell Physiol 2014;229:10-6. [PubMed]
- Rosti V, Bonetti E, Bergamaschi G, et al. High frequency of endothelial colony forming cells marks a non-active myeloproliferative neoplasm with high risk of splanchnic vein thrombosis. PLoS One 2010;5:e15277. [Crossref] [PubMed]
- Piaggio G, Rosti V, Corselli M, et al. Endothelial colony-forming cells from patients with chronic myeloproliferative disorders lack the disease-specific molecular clonality marker. Blood 2009;114:3127-30. [Crossref] [PubMed]
- Teofili L, Martini M, Iachinoto MG, et al. Endothelial progenitor cells are clonal and exhibit the JAK2V617F mutations in a subset of thrombotic patients with Ph-negative myeloproliferative neoplasms. Blood 2011;117:2700-7. [Crossref] [PubMed]
- Torres C, Fonseca AM, Leander M, et al. Circulating endothelial cells in patients with venous thromboembolism and myeloproliferative neoplasms. PLoS One 2013;8:e81574. [Crossref] [PubMed]
- Campanelli R, Rosti V, Fois G, et al. CD14brightCD16low intermediate monocytes expressing Tie2 are increased in the peripheral blood of patients with primary myelofibrosis. Exp Hematol 2014;42:244-6. [Crossref] [PubMed]
- Sozer S, Wang X, Zhang W, et al. Circulating angiogenic monocyte progenitor cells are reduced in JAK2V617F high allele burden myeloproliferative disorders. Blood Cells Mol Dis 2008;41:284-91. [Crossref] [PubMed]
- Massa M, Rosti V, Ramajoli I, et al. Circulating CD34+, CD133+, and vascular endothelial growth factor receptor 2-positive endothelial progenitor cells in myelofibrosis with myeloid metaplasia. J Clin Oncol 2005;23:5688-95. [Crossref] [PubMed]
- Rose JA, Erzurum S, Asosingh K. Biology and flow cytometry of proangiogenic hematopoietic progenitors cells. Cytometry A 2015;87:5-19. [Crossref] [PubMed]
- Wara AK, Croce K, Foo S, et al. BM-derived CMPs and GMPs represent highly functional proangiogenic cells: implications for ischemic cardiovascular disease. Blood 2011;118:6461-4. [Crossref] [PubMed]
- Kopp H G, Ramos CA, Rafii S. Contribution of endothelial progenitors and proangiogenic hematopoietic cells to vascularization of tumor and ischemic tissue. Curr Opin Hematol 2006;13:175-81. [Crossref] [PubMed]
- Gill M, Dias S, Hattori K, et al. Vascular trauma induces rapid but transient mobilization of VEGFR2 (+) AC133 (+) endothelial precursor cells. Circ Res 2001;88:167-74. [Crossref] [PubMed]
- Yoon CH, Hur J, Park KW, et al. Synergistic neovascularization by mixed transplantation of early endothelial progenitor cells and late outgrowth endothelial cells: the role of angiogenic cytokines and matrix metalloproteinases. Circulation 2005;112:1618-27. [Crossref] [PubMed]
- Yoder MC. Human endothelial progenitor cells. Cold Spring Harb Perspect Med 2012;2:a006692. [Crossref] [PubMed]
- Lin Y, Weisdorf DJ, Solovey A, et al. Origins of circulating endothelial cells and endothelial outgrowth from blood. J Clin Invest 2000;105:71-7. [Crossref] [PubMed]
- Ingram DA, Mead LE, Tanaka H, et al. Identification of a novel hierarchy of endothelial progenitor cells using human peripheral and umbilical cord blood. Blood 2004;104:2752-60. [Crossref] [PubMed]
- Ingram DA, Caplice NM, Yoder MC. Unresolved questions, changing definitions, and novel paradigms for defining endothelial progenitor cells. Blood 2005;106:1525-31. [Crossref] [PubMed]
- Yoder MC, Mead LE, Prater D, et al. Redefining endothelial progenitor cells via clonal analysis and hematopoietic stem/progenitor cell principals. Blood 2007;109:1801-9. [Crossref] [PubMed]
- Timmermans F, Van Hauwermeiren F, De Smedt M, et al. Endothelial outgrowth cells are not derived from CD133+ cells or CD45+ hematopoietic precursors. Arterioscler Thromb Vasc Biol 2007;27:1572-9. [Crossref] [PubMed]
- Tura O, Skinner EM, Barclay GR, et al. Late outgrowth endothelial cells resemble mature endothelial cells and are not derived from bone marrow. Stem Cells 2013;31:338-48. [Crossref] [PubMed]
- Patel J, Wong HY, Wang W, et al. Self-renewal and high proliferative colony forming capacity of late-outgrowth endothelial progenitors is regulated by cyclin-dependent kinase inhibitors driven by notch signaling. Stem Cells 2016;34:902-12. [Crossref] [PubMed]
- Ikutomi M, Sahara M. Diverse contribution of bone marrow-derived late-outgrowth endothelial progenitor cells to vascular repair under pulmonary arterial hypertension and arterial neointimal formation. J Mol Cell Cardiol 2015;86:121-35. [Crossref] [PubMed]
- Kim SW, Jin HL, Kang SM, et al. Therapeutic effects of late outgrowth endothelial progenitor cells or mesenchymal stem cells derived from human umbilical cord blood on infarct repair. Int J Cardiol 2016;203:498-507. [Crossref] [PubMed]
- Gunsilius E, Duba HC, Petzer AL, et al. Evidence from a leukemia model for maintenance of vascular endothelium by bone marrow-derived endothelial cells. Lancet 2000;355:1688-91. [Crossref] [PubMed]
- Wu J, Huang L, Huang M, et al. Dominant contribution of malignant endothelial cells to endotheliopoiesiss in chronic myeloid leukemia. Exp Hematol 2009;37:87-91. [Crossref] [PubMed]
- Otten J, Schultze A, Schafhausen P, et al. Blood outgrowth endothelial cells from chronic myeloid leukaemia patients are BCR/ABL1 negative. Br J Haematol 2008;142:115-8. [Crossref] [PubMed]
- Fang B, Zheng C, Liao L, et al. Identification of human chronic myelogenous leukemia progenitor cells with hemangioblastic characteristics. Blood 2005;105:2733-40. [Crossref] [PubMed]
- Xishan Z, Xu Z, Lawei Y, et al. Hemangioblastic characteristics of cancer stem cells in chronic myeloid leukemia. Clin Lab 2012;58:607-13. [PubMed]
- Lundberg LG, Lerner R, Sundelin P, et al. Bone marrow in polycythemia vera, chronic myelocytic leukemia, and myelofibrosis has an increased vascularity. Am J Pathol 2000;157:15-9. [Crossref] [PubMed]
- Mahadevan KK, Basu D, Kumar S. Does CD34 staining reflect the angiogenic process in the bone marrow? An analysis of a series of chronic myeloid leukemia patients. J Clin Diagn Res 2014;8:FC04-7. [PubMed]
- Taverna S, Amodeo L, Saieva L, et al. Exosomal shutting of miR-126 in endothelial cells modulates adhesive and migratory abilities of chronic myelogenous leukemia cells. Mol Cancer 2014;13:169. [Crossref] [PubMed]
- Taverna S, Flugy A, Saieva L, et al. Role of exosomes released by chronic myelogenous leukemia cells in angiogenesis. Int J Cancer 2012;130:2033-43. [Crossref] [PubMed]
- Taverna S, Fontana S, Monteleone F, et al. Curcumin modulates chronic myelogenous leukemia exosomes composition and affects angiogenic phenotype, via exosomal miR-21. Oncotarget 2016. [Epub ahead of print]. [PubMed]
- Ramos TL, Sanchez-Abarca LI, Lòpez-Ruano G, et al. Do endothelial cells belong to the primitive stem cell clone in CML? Role of extracellular vesicles. Leuk Res 2015;39:921-4. [Crossref] [PubMed]
- Cai J, Han Y, Ren H, et al. Extracellular vesiscle-mediated transfer of donor genomic DNA to recipient cells is a novel mechanism for genetic influence between cells. J Mol Cell Biol 2013;5:227-38. [Crossref] [PubMed]
- Cai J, Wu G, Jan X, et al. Transferred BCR/ABL DNA from K562 extracellular vesicles causes chronic myeloid leukemia in immunodeficient mice. PLoS One 2014;9:e105200. [Crossref] [PubMed]
- Sozer S, Fiel MI, Schiano T, et al. The presence of JAK2V617F mutation in the liver endothelial cells of patients with Budd-Chiari syndrome. Blood 2009;113:5246-9. [Crossref] [PubMed]
- Rosti V, Villani L, Riboni R, et al. Spleen endothelial cells from patients with myelofibrosis harbor the JAK2V617F mutation. Blood 2013;121:360-8. [Crossref] [PubMed]
- Gianelli U, Vener C, Raviele PR, et al. VEGF expression correlates with microvessel density in Philadelphia chromosome-negative chronic myeloproliferative disorders. Am J Clin Pathol 2007;128:966-73. [Crossref] [PubMed]
- Medinger M, Skoda R, Gratwohl A, et al. Angiogenesis and vascular endothelial growth factor-/receptor expression in myeloproliferative neoplasms: correlation with clinical parameters and JAK2-V617F mutational status. Br J Haematol 2009;146:150-7. [Crossref] [PubMed]
- Boiocchi L, Vener C, Savi F, et al. Increased expression of vascular endothelial growth factor receptor1 correlates with VEGF and microvessel density in Philadelphia chromosome-negative myeloproliferative neoplasms. J Clin Pathol 2011;64:226-31. [Crossref] [PubMed]
- Shirzad R, Shahrabi S, Ahmadzadeh A, et al. Signaling and molecular basis of bone marrow niche angiogenesis in leukemia. Clin Transl Oncol 2016. [Epub ahead of print]. [Crossref] [PubMed]
- Hou HA, Chou WC, Lin LI, et al. Expression of angiopoietins and vascular endothelial growth factors and their clinical significance in acute myeloid leukemia. Leuk Res 2008;32:904-12. [Crossref] [PubMed]
- Padró T, Bieker R, Ruiz S, et al. Overexpression of vascular endothelial growth factor (VEGF) and its cellular receptor KDR (VEGFR-2) in the bone marrow of patients with acute myeloid leukemia. Leukemia 2002;16:1302-10. [Crossref] [PubMed]
- Hiramatsu A, Miwa H, Shikami M, et al. Disease-specific expression of VEGF and its receptors in AML cells: possible autocrine pathway of VEGF/type 1 receptor of VEGF in t(15;17) AML and VEGFD/type 2 receptor of VEGF in t(8;21) AML. Leuk Lymphoma 2006;47:89-95. [Crossref] [PubMed]
- Ter Elst A, Ma B, Scherpen FJ, et al. Repression of vascular endothelial growth factor expression by the runt-related transcription factor 1 in acute myeloid leukemia. Cancer Res 2011;71:2761-71. [Crossref] [PubMed]
- Saulle E, Petronelli A, Pelosi E, et al. PML-RAR alpha induces the downmodulation of HHEX: a key event responsible for the induction of an angiogenetic response. J Hematol Oncol 2016;9:33. [Crossref] [PubMed]
- Kampen KR, Ter Elst A, de Bont ES. Vascular endothelial growth factor signaling in acute myeloid leukemia. Cell Mol Life Sci 2013;70:1307-17. [Crossref] [PubMed]
- Watarai M, Miwa H, Shikami M, et al. Expression of endothelial cell-associated molecules in AML cells. Leukemia 2002;16:112-9. [Crossref] [PubMed]
- Riccioni R, Diverio D, Mariani G, et al. Expression of Tie-2 and other receptors for endothelial growth factors in acute myeloid leukemias is associated with monocytic features of leukemic blasts. Stem Cells 2007;25:1862-71. [Crossref] [PubMed]
- Imai N, Shikami M, Miwa H, et al. t(8;21) acute myeloid leukemia cells are dependent on vascular endothelial growth factor (VEGF)/VEGF receptor type 2 pathway and phosphorylation of AKT. Br J Haematol 2006;135:673-82. [Crossref] [PubMed]
- Coppola S, Narciso L, Feccia T, et al. Enforced expression of KDR receptor promotes proliferation, survival and megakaryocytic differentiation of TF1 progenitor cell line. Cell Death Differ 2006;13:61-74. [Crossref] [PubMed]
- Padró T, Ruiz S, Bieker R, et al. Increased angiogenesis in the bone marrow of patients with acute myeloid leukemia. Blood 2000;95:2637-44. [PubMed]
- Hussong JW, Rodgers GM, Shami PJ. Evidence of increased angiogenesis in patients with acute myeloid leukemia. Blood 2000;95:309-13. [PubMed]
- Kini AR, Peterson LA, Tallman MS, et al. Angiogenesis in acute promyelocytic leukemia: induction by vascular endothelial growth factor and inhibition by all-trans retinoic acid. Blood 2001;97:3919-24. [Crossref] [PubMed]
- Zhang J, Ye Y, Ma D, et al. Cross-talk between leukemic and endothelial cells promotes angiogenesis by VEGF activation of the Notch/DLL4 pathway. Carcinogenesis 2013;34:667-77. [Crossref] [PubMed]
- Hatfield K, Øyan AM, Ersvaer E, et al. Primary human acute myeloid leukaemia cells increase the proliferation of microvascular endothelial cells through the release of soluble mediators. Br J Haematol 2009;144:53-68. [Crossref] [PubMed]
- Hatfield KJ, Evensen L, Reikvam H, et al. Soluble mediators released by acute myeloid leukemia cells increase capillary-like networks. Eur J Haematol 2012;89:478-90. [Crossref] [PubMed]
- Hatfield K, Ryningen A, Carbascio M, et al. Microvascular endothelial cells increase proliferation and inhibit apoptosis of native human acute myelogenous leukemia blasts. Int J Cancer 2006;119:2313-21. [Crossref] [PubMed]
- Bosse RC, Wasserstrom B, Meacham A, et al. Chemosensitizing AML cells by targeting bone marrow endothelial cells. Exp Hematol 2016;44:363-377.e5. [Crossref] [PubMed]
- Pizzo RJ, Azadniv M, Guo N, et al. Phenotypic, genotypic, and functional characterization of normal and acute myeloid leukemia-derived marrow endothelial cells. Exp Hematol 2016;44:378-89. [Crossref] [PubMed]
- Shih TT, Hou HA, Liu CY, et al. Bone marrow angiogenesis magnetic resonance imaging in patients with acute myeloid leukemia: peak enhancement ratio in an independent predictor for overall survival. Blood 2009;113:3161-7. [Crossref] [PubMed]
- Cogle CR, Goldman DC, Malambayan GJ, et al. Functional integration of acute myeloid leukemia into the vascular niche. Leukemia 2014;28:1978-87. [Crossref] [PubMed]
- Cogle CR, Bosse RC, Brewer T, et al. Acute myeloid leukemia in the vascular niche. Cancer Lett 2015. [Epub ahead of print]. [Crossref] [PubMed]
- Pruneri G, Bertolini F, Soligo D, et al. Angiogenesis in myelodysplastic syndromes. Br J Cancer 1999;81:1398-401. [Crossref] [PubMed]
- Aguayo A, Kantarjian H, Manshouri T, et al. Angiogenesis in acute and chronic leukemias and myelodysplastc syndromes. Blood 2000;96:2240-5. [PubMed]
- Korkolopoulou P, Apostolidou E, Pavlopoulos PM, et al. Prognostic evaluation of the microvascular network in myelodysplastic syndromes. Leukemia 2001;15:1369-76. [Crossref] [PubMed]
- Alexandrakis MG, Passam FH, Pappa CA, et al. Relation between bone marrow angiogenesis and serum levels of angiogenin in patients with myelodysplastic syndromes. Leuk Res 2005;29:41-6. [Crossref] [PubMed]
- Lundberg LG, Hellstrom-Lindberg E, Kanter-Lewensohn L, et al. Angiogenesis in relation to clinical stage, apoptosis and prognostic score in myelodysplastic syndromes. Leuk Res 2006;30:247-53. [Crossref] [PubMed]
- Madry K, Dwilewicz-Trojaczek J, Suleiman W, et al. Angiogenesis in bone marrow of myelodysplastic syndrome patients. Pol Arch Med Wewn 2007;117:25-30. [PubMed]
- Keith T, Araki Y, Ohyaqi M, et al. Regulation of angiogenesis in the bone marrow of myelodysplastic syndromes transforming to overt leukemia. Br J Haematol 2007;137:206-15. [Crossref] [PubMed]
- Cheng CL, Hou HA, Jhuang JY, et al. High bone marrow angiopoietin-1 expression is an independent poor prognostic factor for survival in patients with myelodysplastic syndromes. Brit J Cancer 2011;105:975-82. [Crossref] [PubMed]
- Della Porta MG, Malcovati L, Rigolin GM, et al. Immunophenotypic, cytogenetic and functional characterization of circulating endothelial cells in myelodysplastic syndromes. Leukemia 2008;22:530-7. [Crossref] [PubMed]
- Teofili L, Martini M, Nuzzolo ER, et al. Endothelial progenitor cell dysfunction in myelodysplastic syndromes: possible contribution of a defective vascular niche to myelodysplasia. Neoplasia 2015;17:401-9. [Crossref] [PubMed]
- Vacca A, Ria R, Reale A, et al. Angiogenesis in multiple myeloma. Chem Immunol Allergy 2014;99:180-96. [Crossref] [PubMed]
- Vacca A, Ribatti D, Presta M, et al. Bone marrow neovascularization, plasma cell angiogenic potential, and matrix metalloproteinase-2 secretion paralell progression of human multiple myeloma. Blood 1999;93:3064-73. [PubMed]
- Rajkumar SV, Mesa RA, Fonseca R, et al. Bone marrow angiogenesis in 400 patients with monoclonal gammopathy of undetermined significance, multiple myeloma, and primary amyloidosis. Clin Cancer Res 2002;8:2210-6. [PubMed]
- Rajkumar SV, Leong T, Roche PC, et al. Prognostic value of bone marrow angiogenesis in multiple myeloma. Clin Cancer Res 2000;6:3111-6. [PubMed]
- Kumar S, Witzig TE, Timm M, et al. Bone marrow angiogenic ability and expression of angiogenic cytokines in myeloma: evidence favoring loss of marrow angiogenesis inhibitory activity with disease progression. Blood 2004;104:1159-65. [Crossref] [PubMed]
- Dankbar B, Padrò T, Leo R, et al. Vascular endothelial growth factor and interleukin-6 in paracrine tumor-stromal cell inetractions in mulktiple myeloma. Blood 2000;95:2630-6. [PubMed]
- Giuliani N, Colla S, Lazzaretti M, et al. Proangiogenic properties of human myeloma cells: production of angiopoietin-1 and its potential relationship to myeloma-induced angiogenesis. Blood 2003;102:638-45. [Crossref] [PubMed]
- Colla S, Marandi F, Lazzaretti M, et al. Human myeloma cells express the bone regulating gene Runx2/Cbfa1 and produce osteopontin that is involved in angiogenesis in multiple myeloma patients. Leukemia 2005;19:2166-76. [Crossref] [PubMed]
- Kocemba KA, van Andel H, de Haan-Kramer A, et al. The hypoxia target adrenomedullin is aberrantly expressed in multiple myeloma and promotes angiogenesis. Leukemia 2013;27:1729-37. [Crossref] [PubMed]
- Vacca A, Ria R, Semeraro F, et al. Endothelial cells in the bone marrow of patients with multiple myeloma. Blood 2003;102:3340-8. [Crossref] [PubMed]
- Zhang H, Vakil V, Branistein M, et al. Circulating endothelial progenitor cells in multiple myeloma: implications and significance. Blood 2005;105:3286-94. [Crossref] [PubMed]
- Rigolin GM, Fraulini C, Ciccone M, et al. Neoplastic circulating endothelial cells in multiple myeloma 13q14 deletion. Blood 2006;107:2531-5. [Crossref] [PubMed]
- Roccaro AM, Sacco A, Maisu P, et al. BM mesenchymal stromal cell-derived exosomes facilitate multiple myeloma progression. J Clin Invest 2013;123:1542-55. [Crossref] [PubMed]
- Umezu T, Tadokoro H, Azuma K, et al. Exosomal miR-135b shed from hypoxic mutiple myeloma cells enhances angiogenesis by targeting factor-inhibiting HIF-1. Blood 2014;124:3748-57. [Crossref] [PubMed]
- Wang J, De Vierman K, Faict S, et al. Multiple myeloma exosomes establish a favourable bone marrow microenvironment with enhanced angiogenesis and immunosuppression. J Pathol 2016;239:162-73. [Crossref] [PubMed]
- Moschetta M, Mishima Y, Kawano Y, et al. Targeting vasculogenesis in multiple myeloma. Leukemia 2016;30:1103-15. [Crossref] [PubMed]
Cite this article as: Testa U, Saulle E, Castelli G, Pelosi E. Endothelial progenitor cells in hematologic malignancies. Stem Cell Investig 2016;3:26.